Lecture No 12 Superconductivity for Accelerators Soren Prestemon
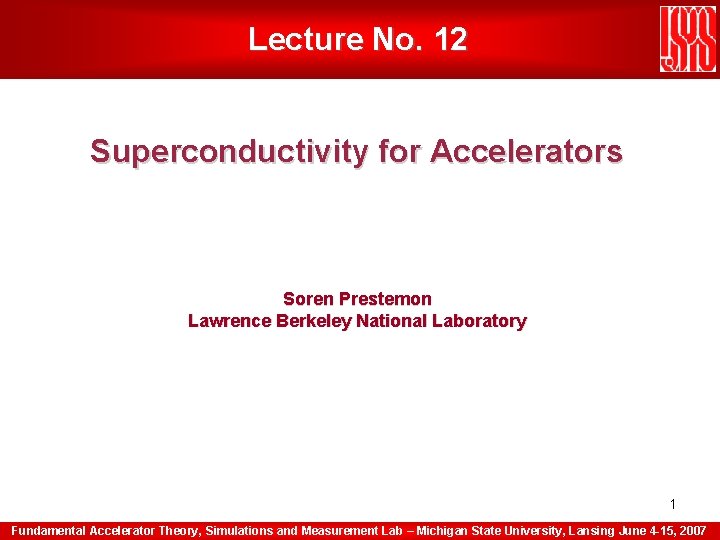
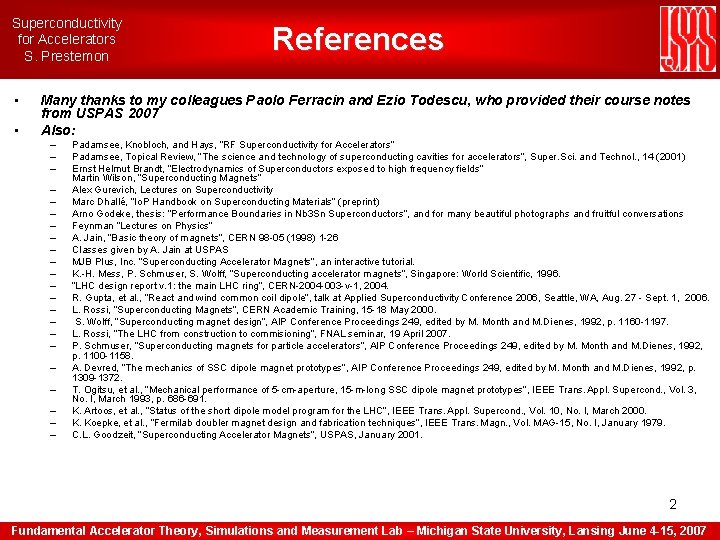
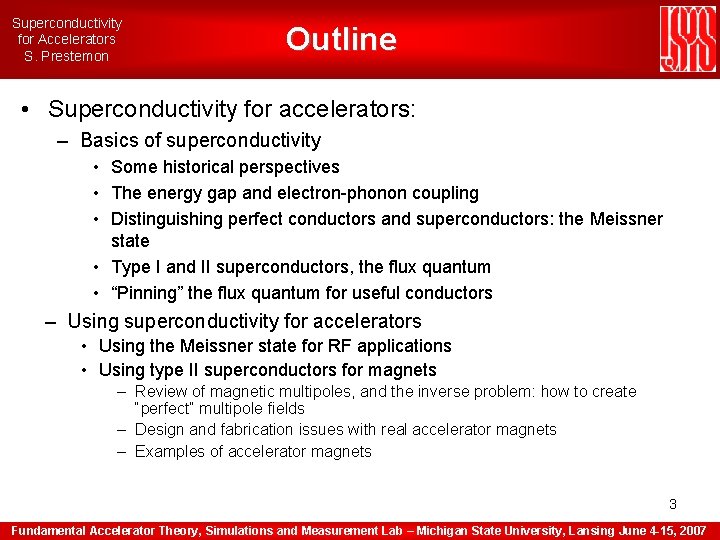
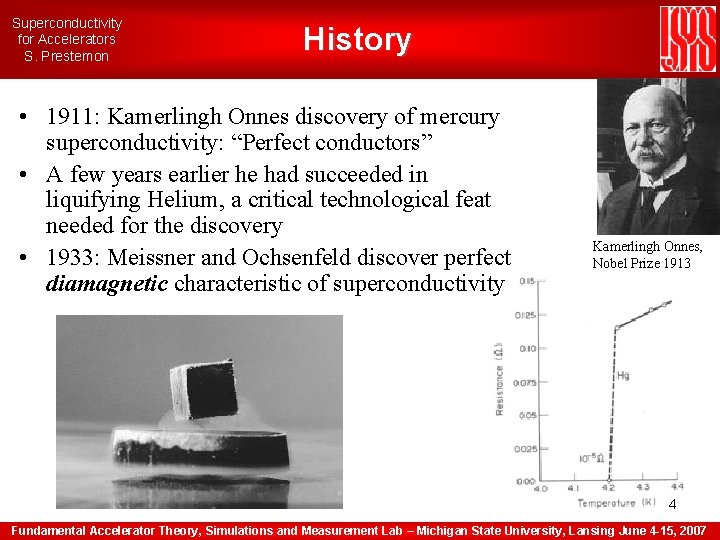
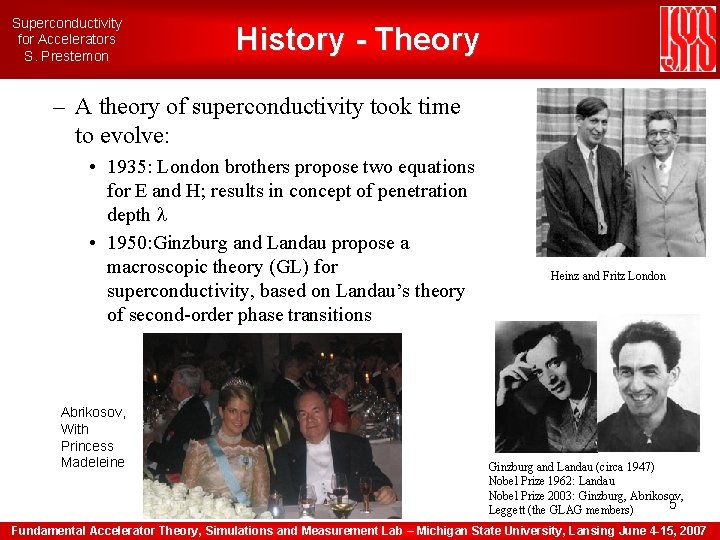
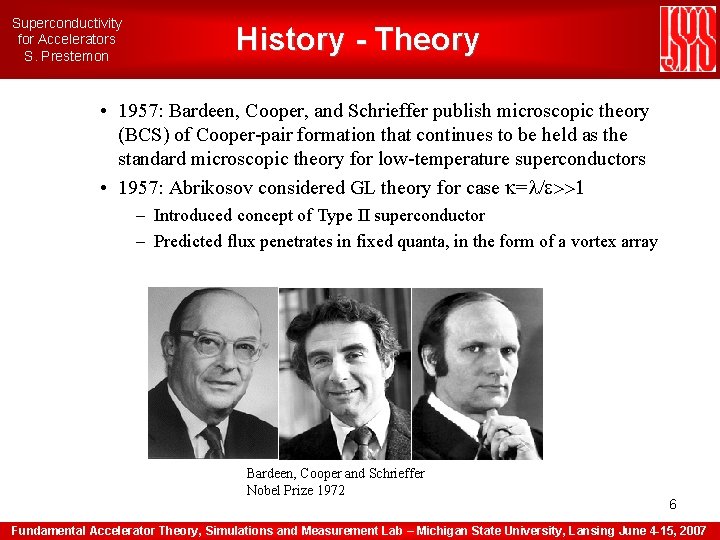
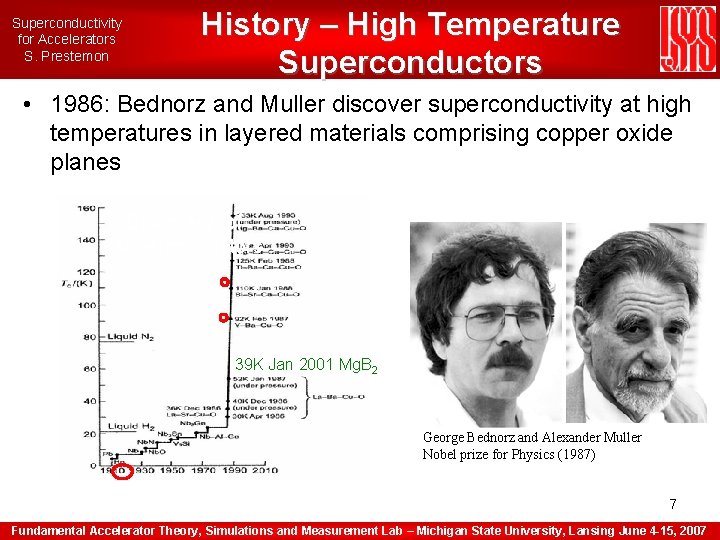
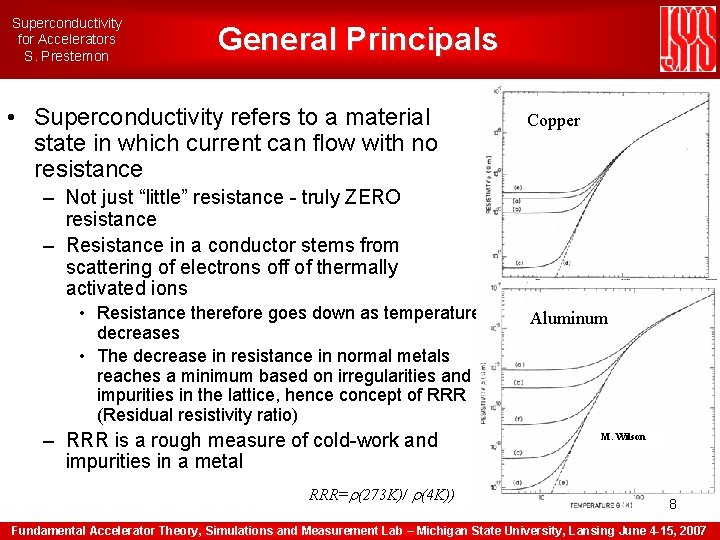
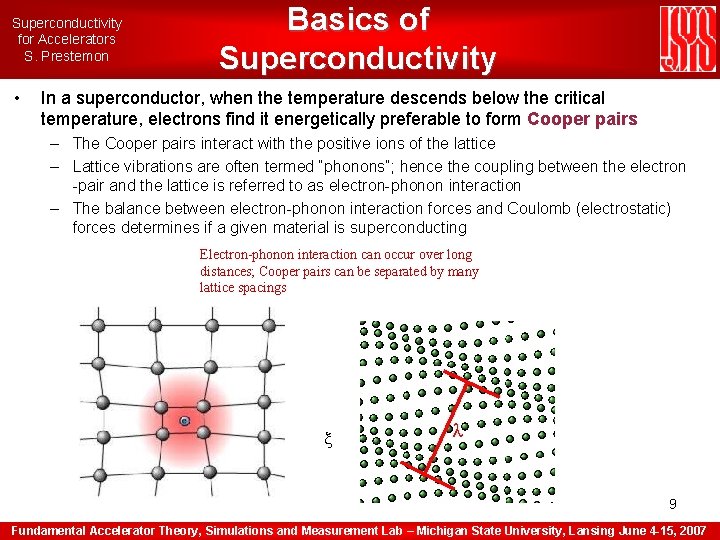
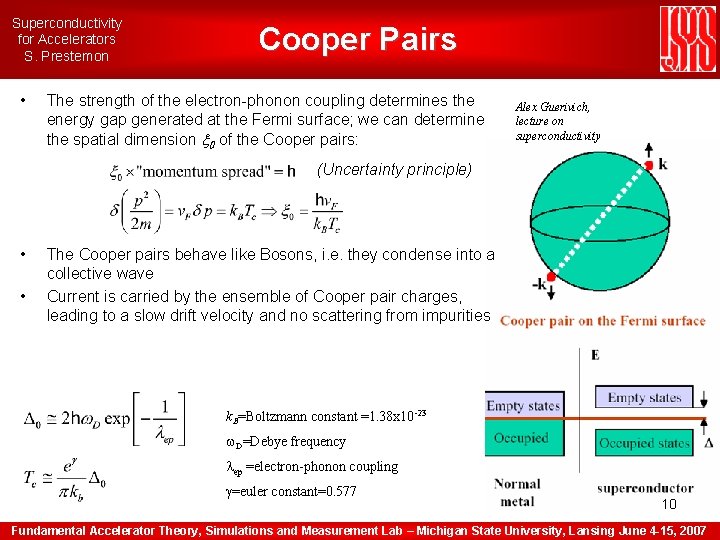
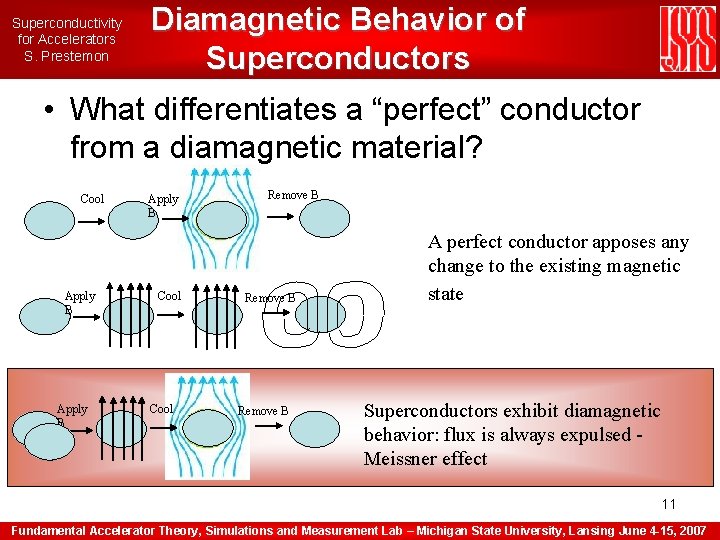
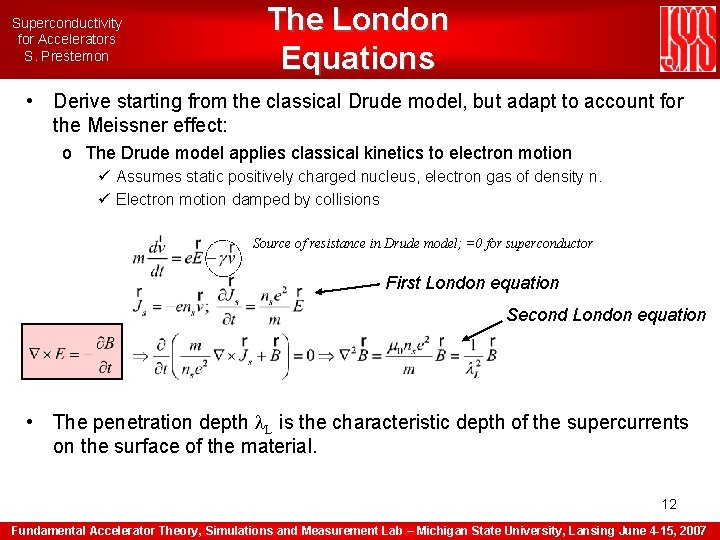
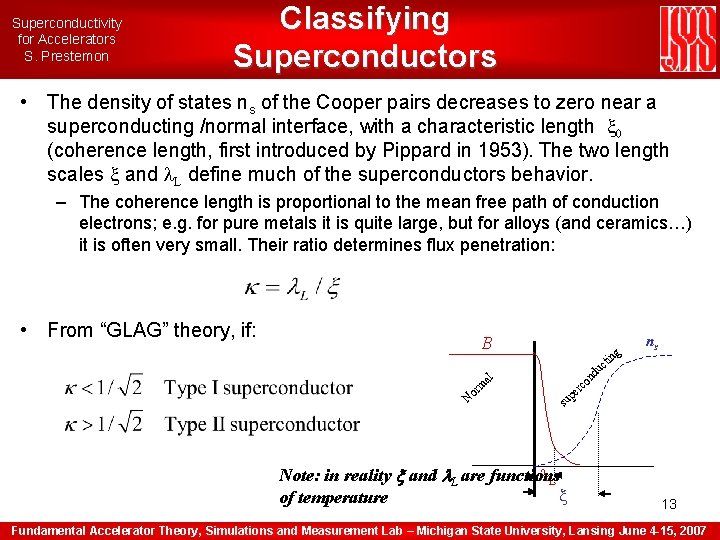
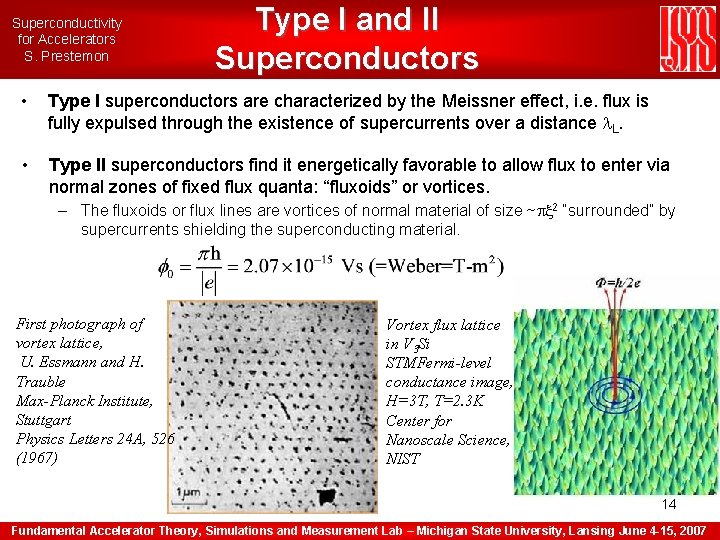
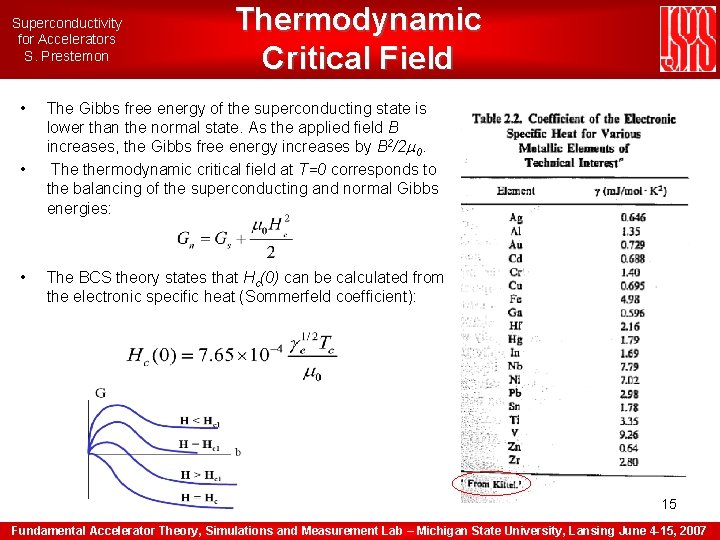
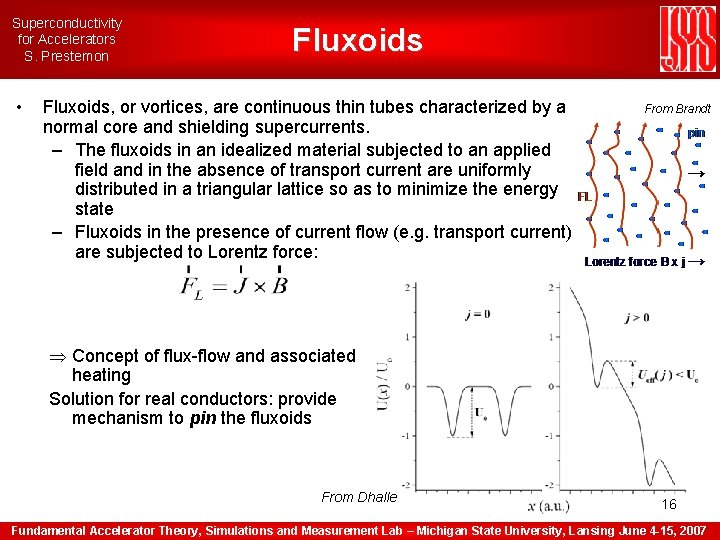
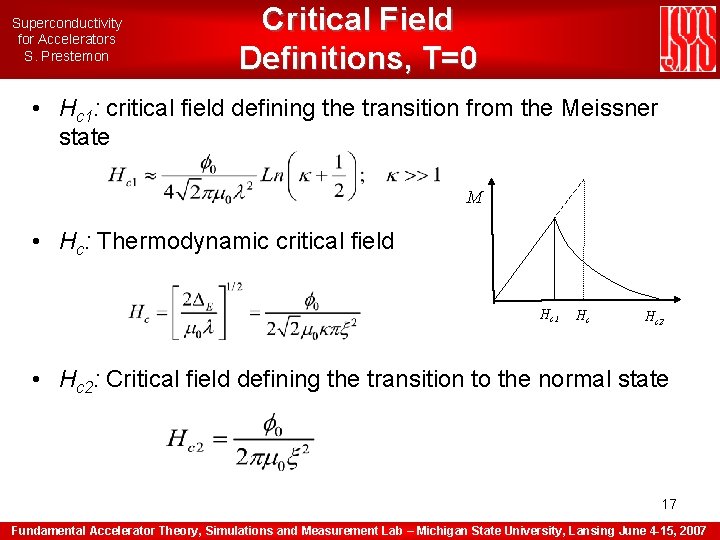
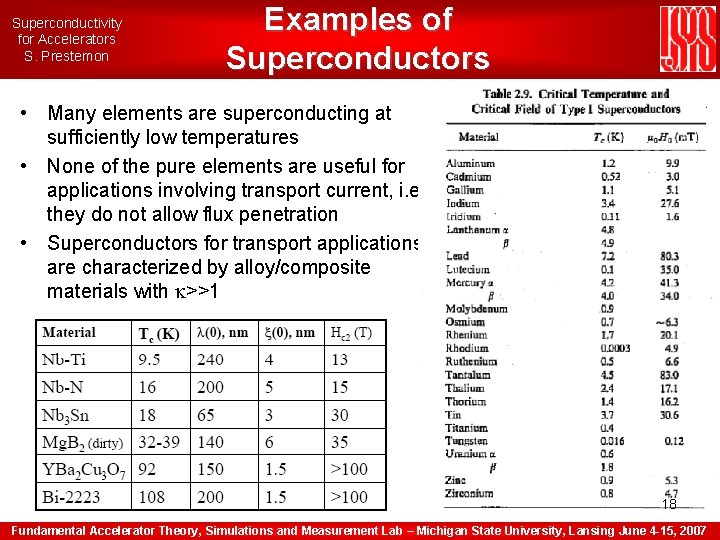
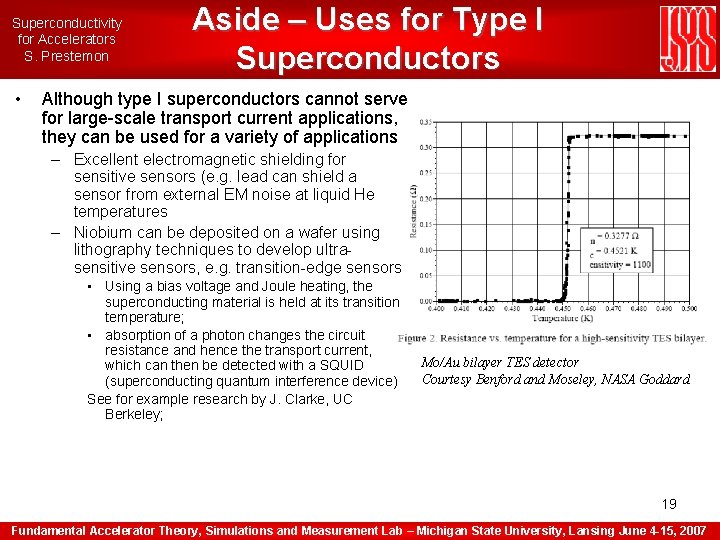
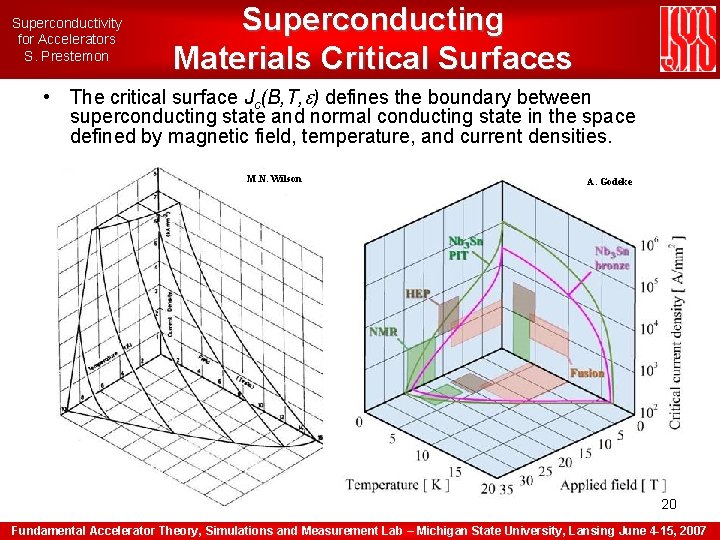
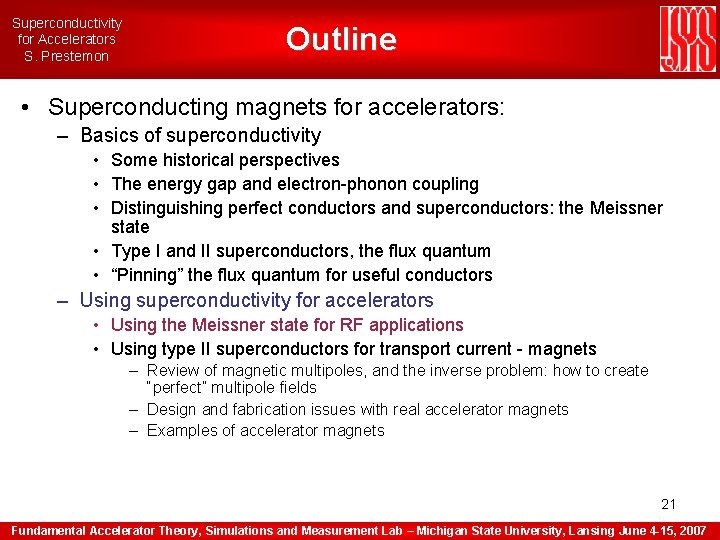
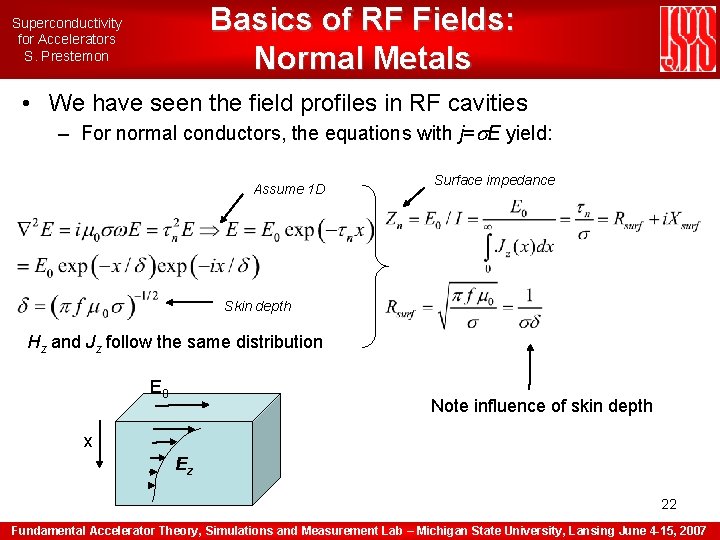
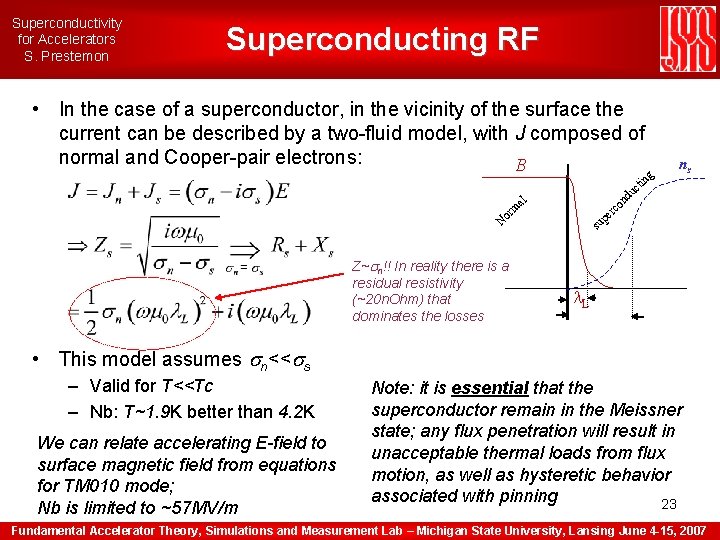
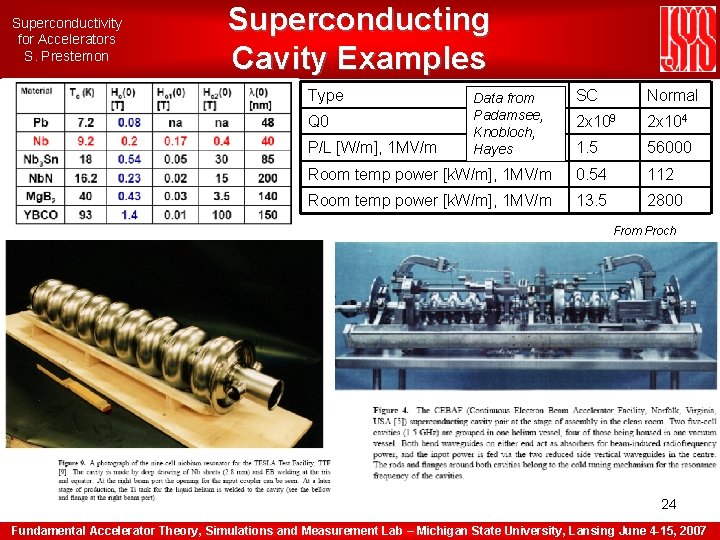
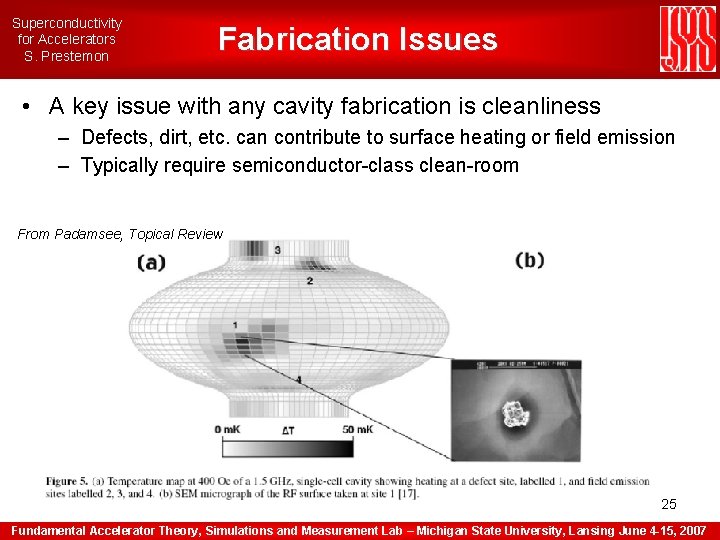
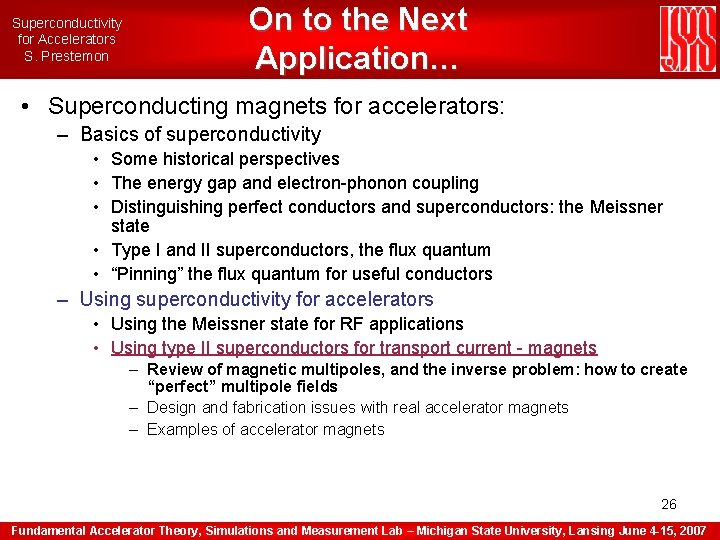
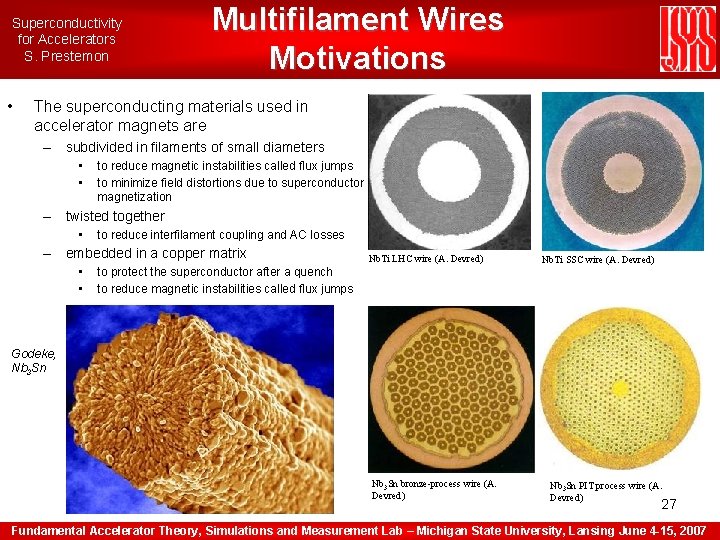
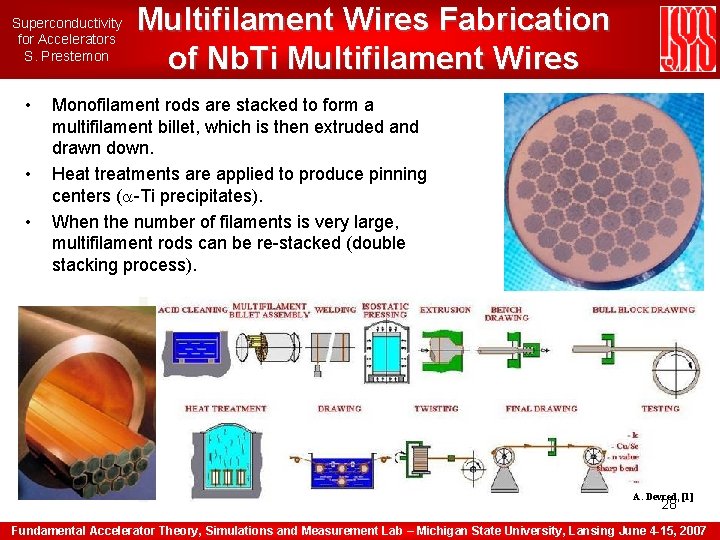
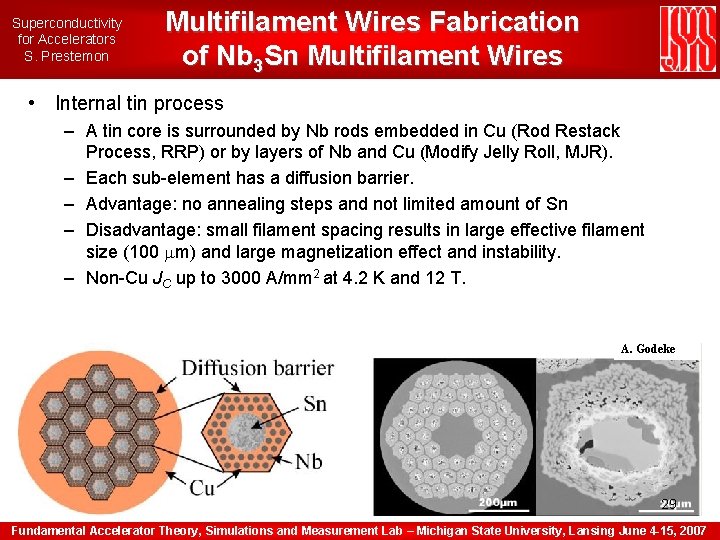
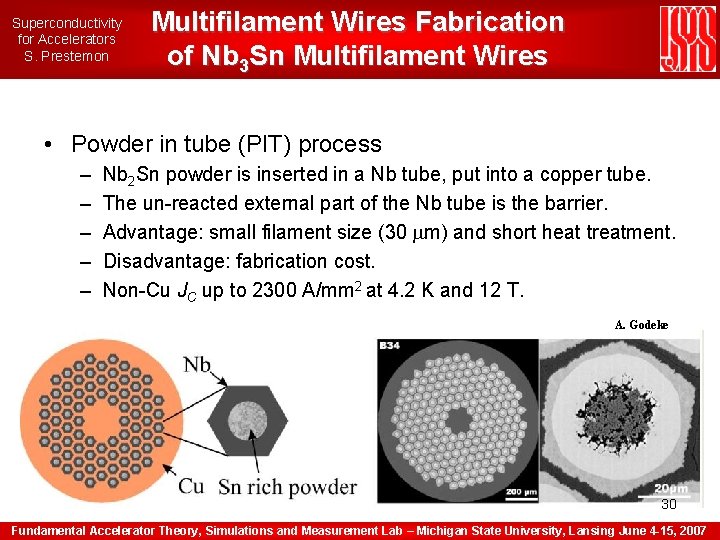
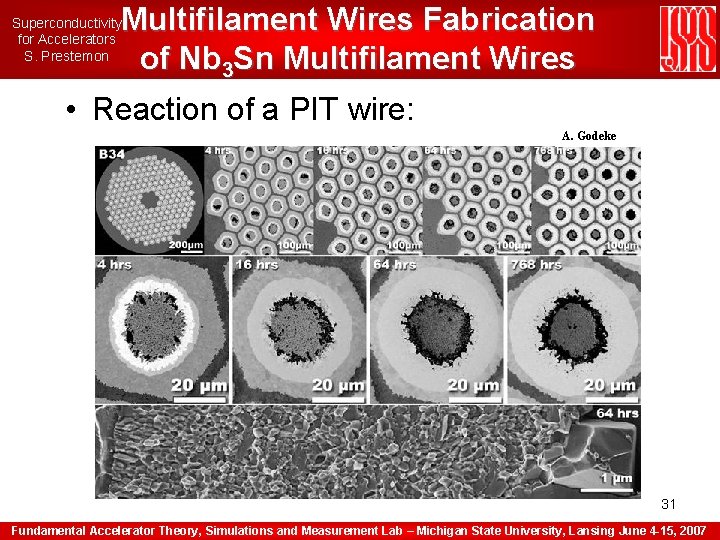
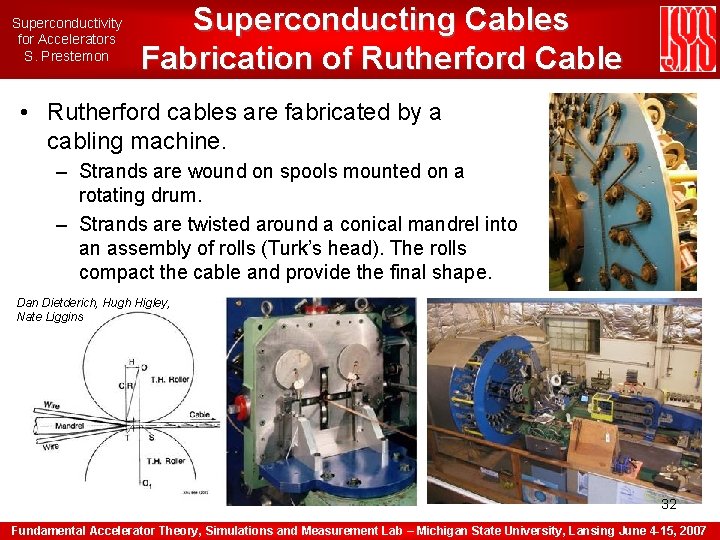
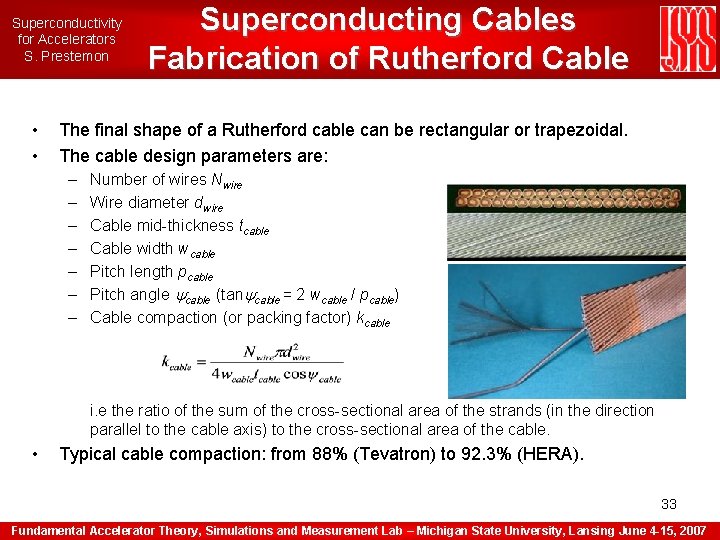
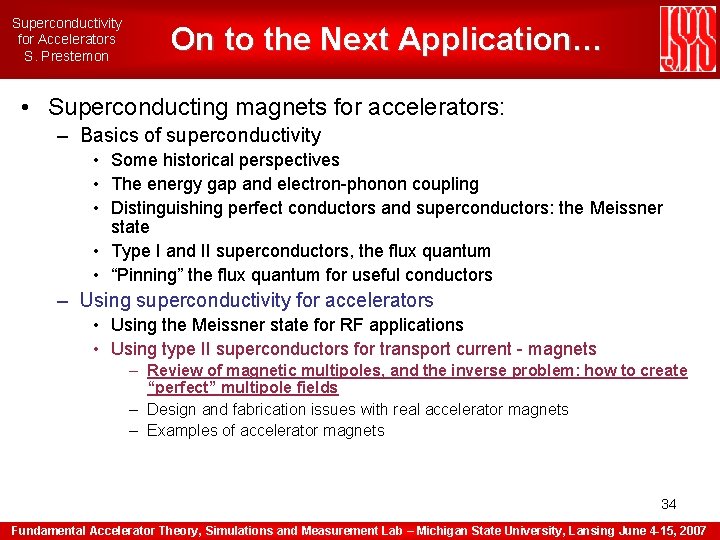
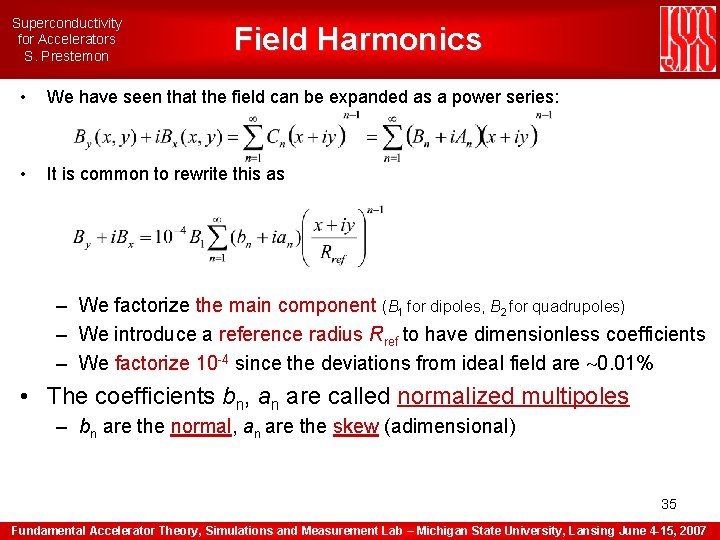
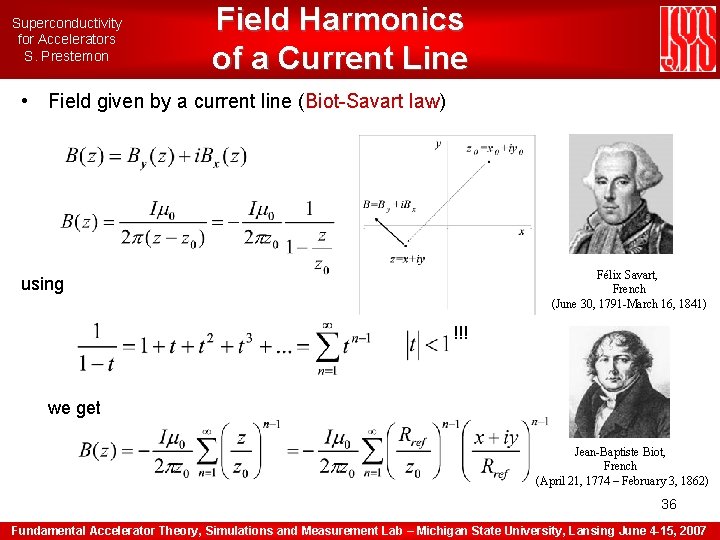
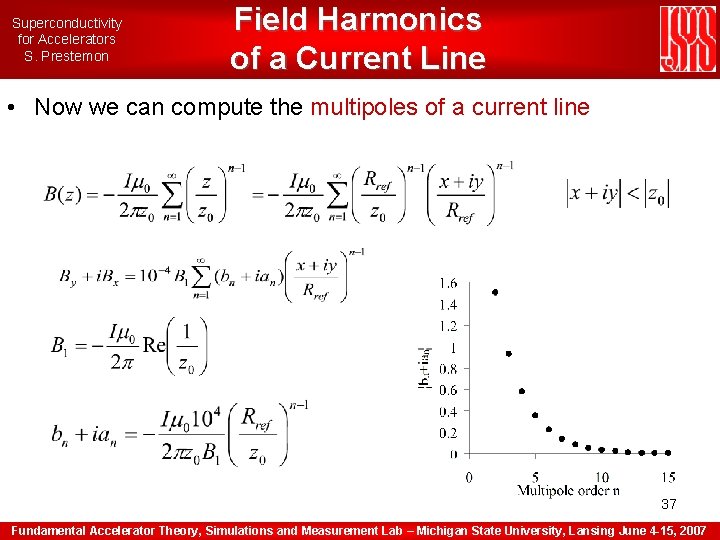
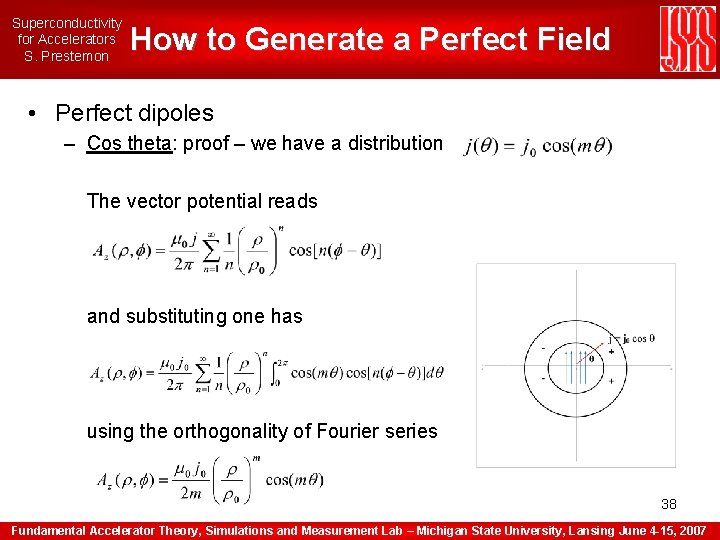
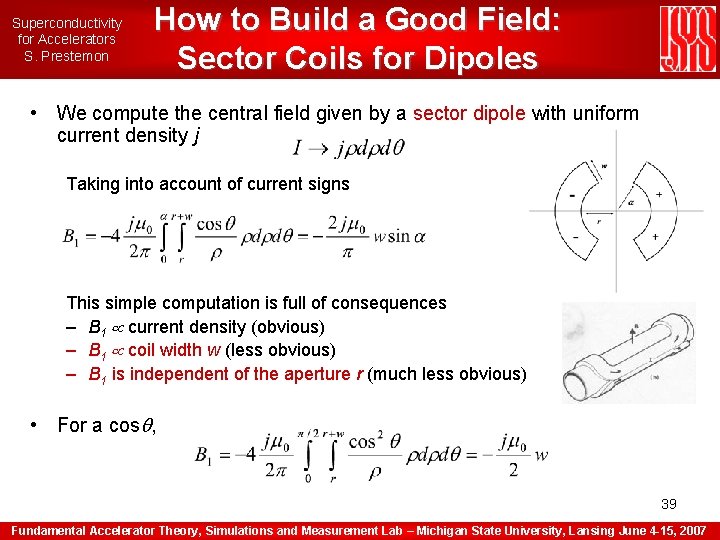
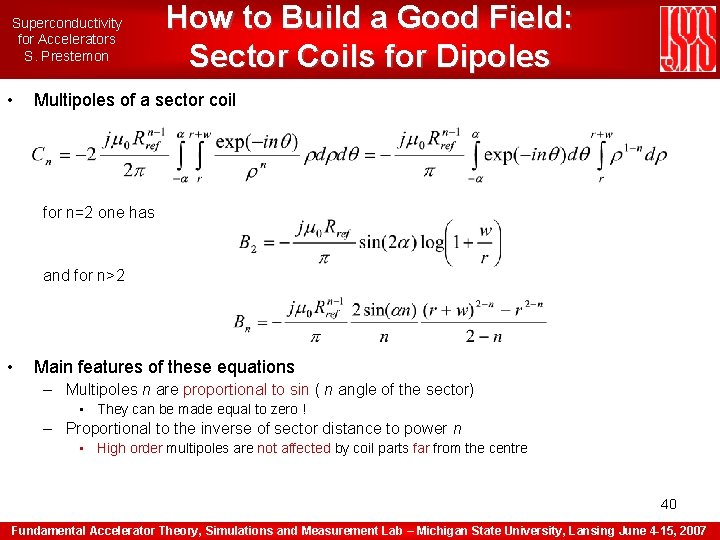
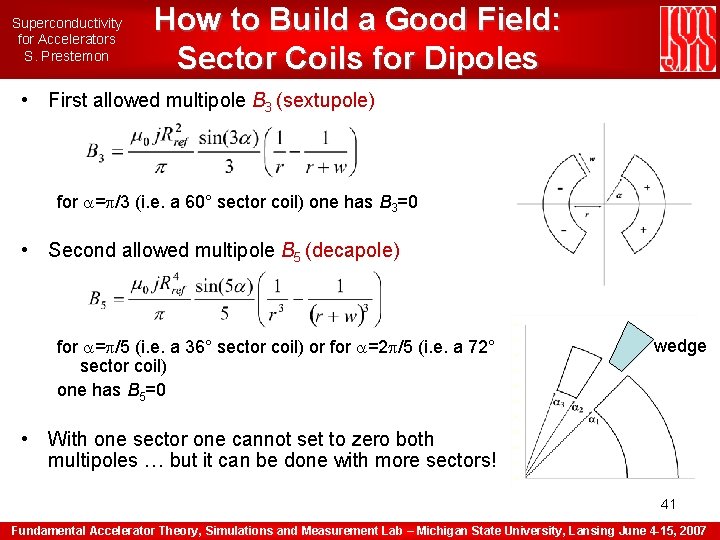
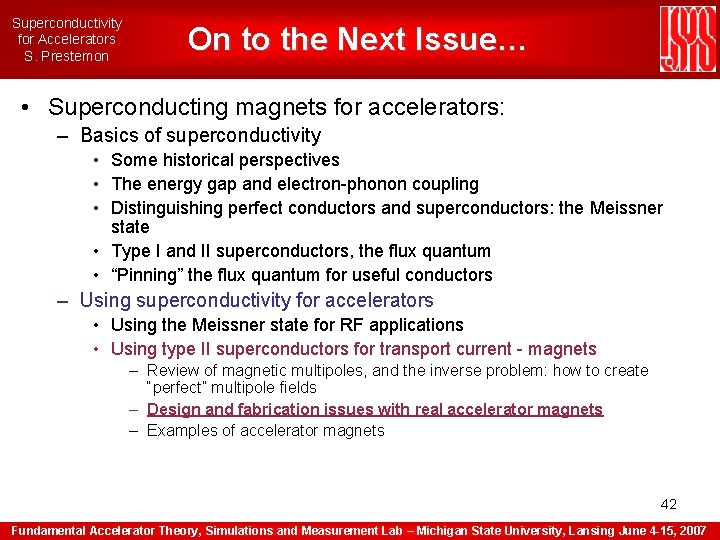
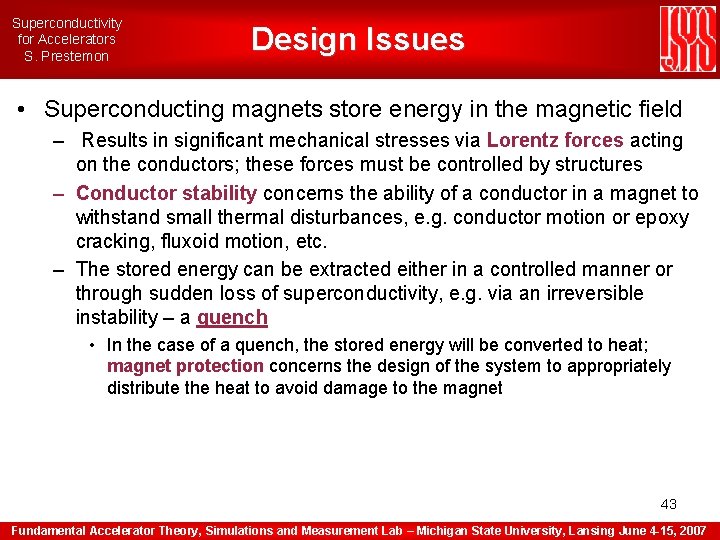
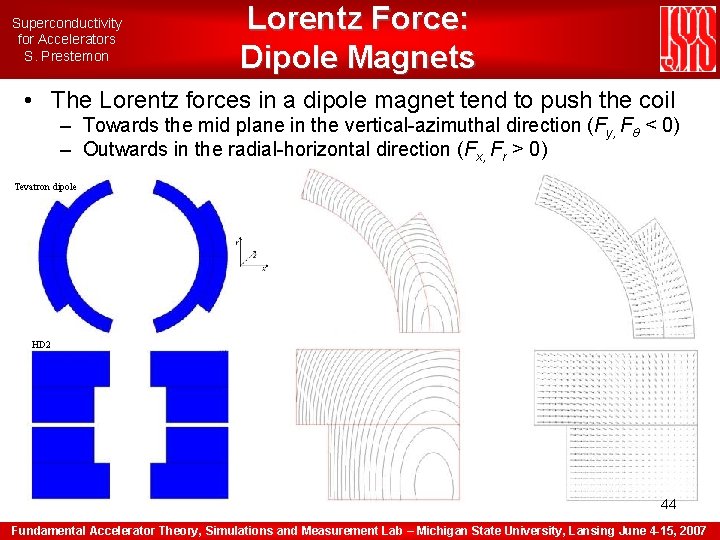
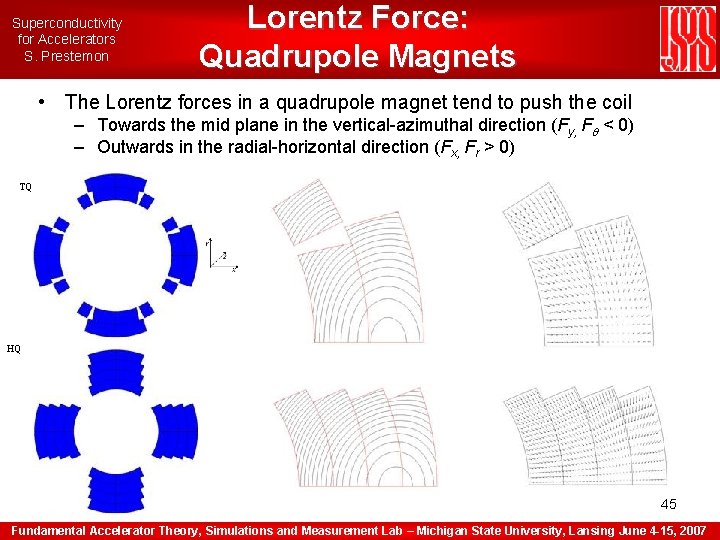
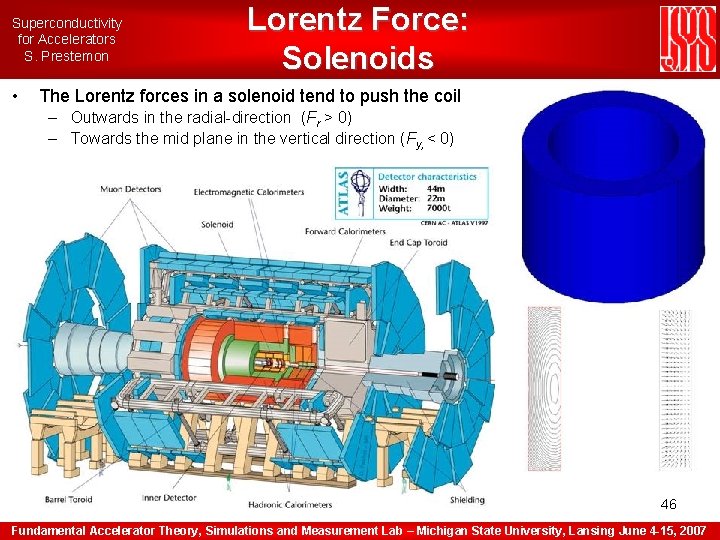
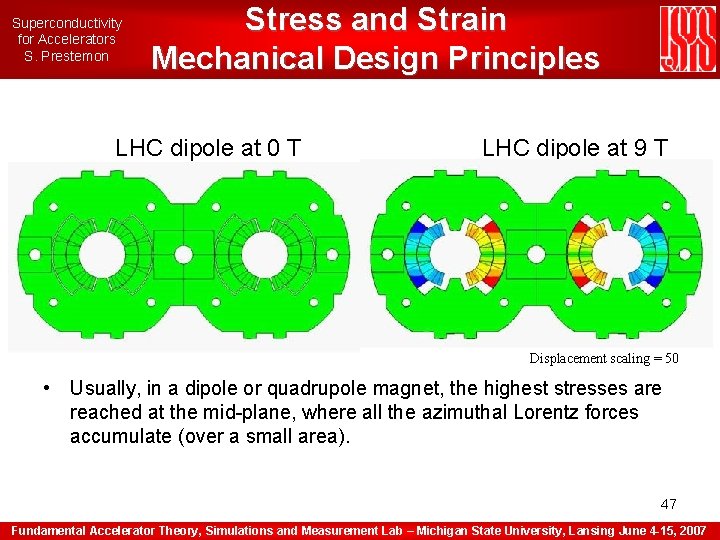
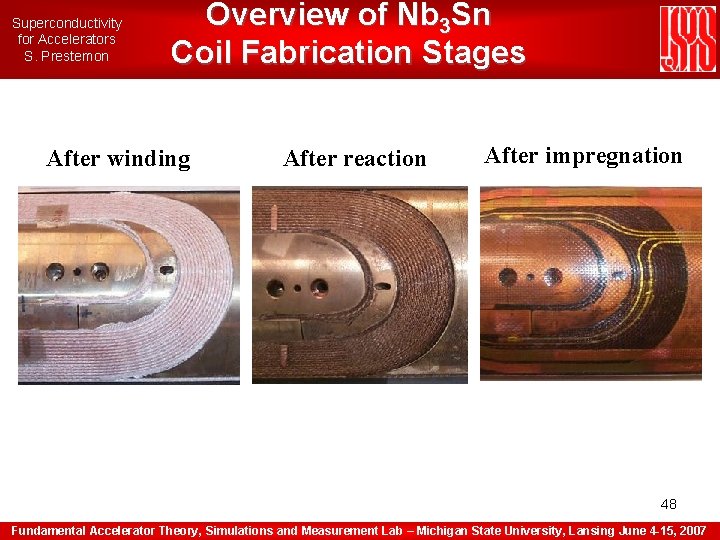
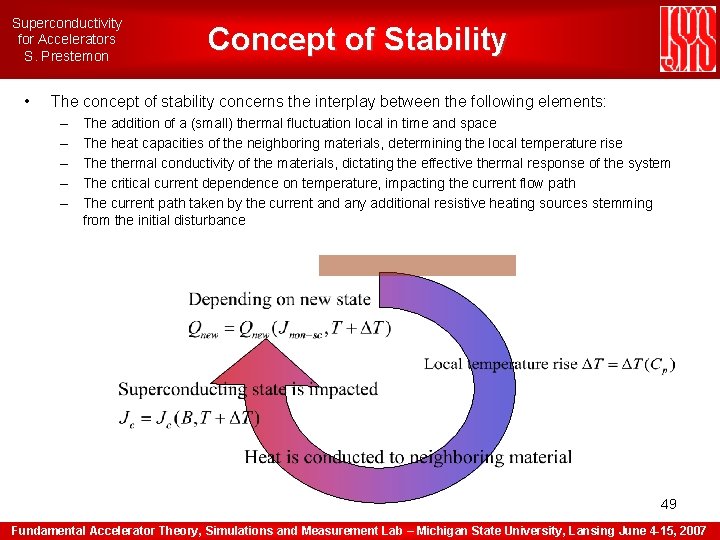
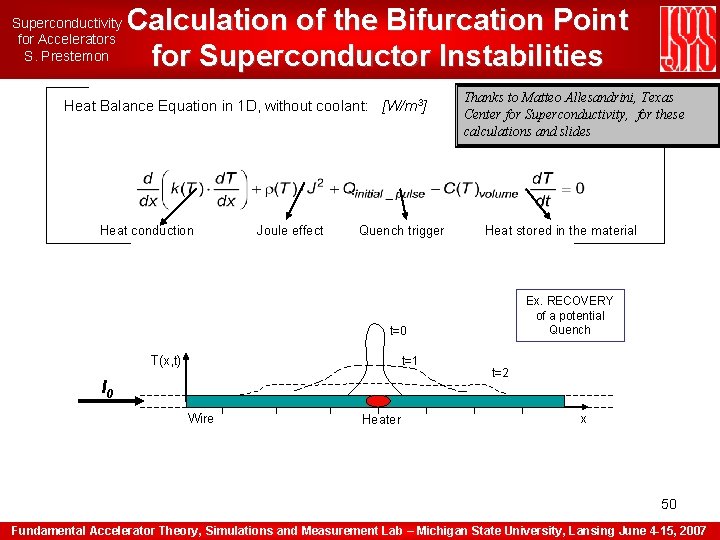
![Superconductivity for Accelerators S. Prestemon Analysis of SQ 02 QUENCH with 1 [m. J] Superconductivity for Accelerators S. Prestemon Analysis of SQ 02 QUENCH with 1 [m. J]](https://slidetodoc.com/presentation_image_h2/31365df123dbc3156dad0acbdf941176/image-51.jpg)
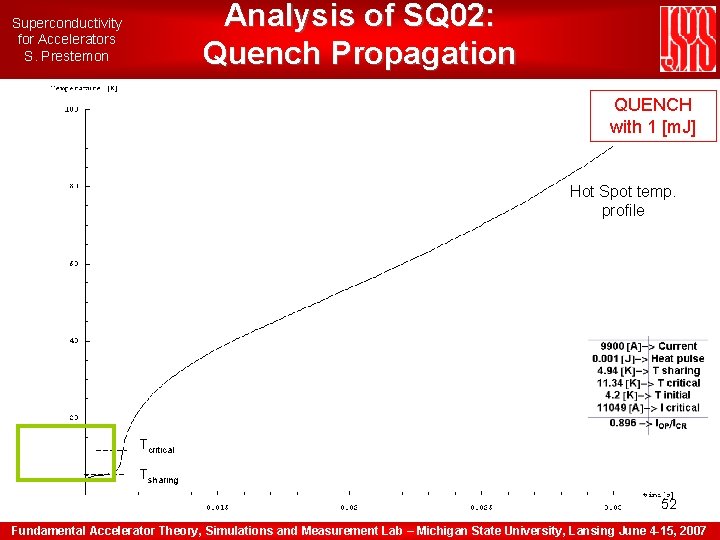
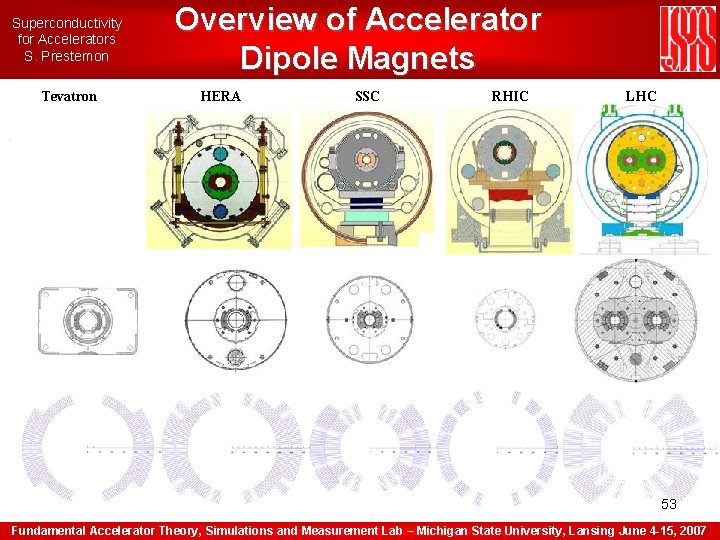
- Slides: 53
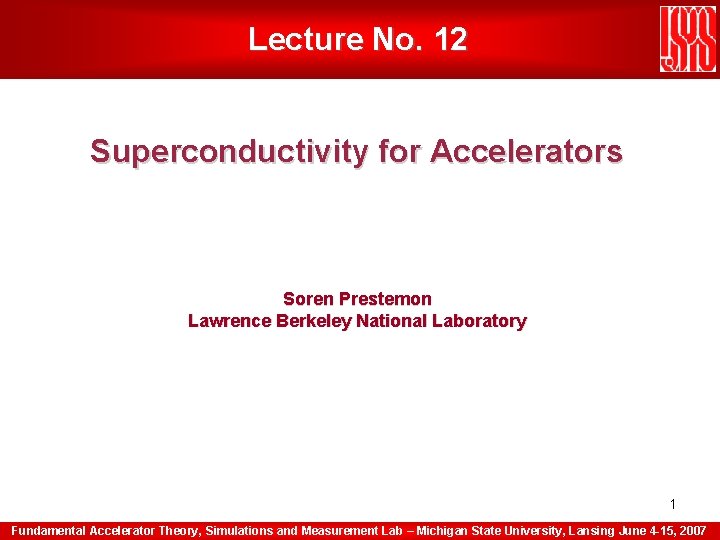
Lecture No. 12 Superconductivity for Accelerators Soren Prestemon Lawrence Berkeley National Laboratory 1 Fundamental Accelerator Theory, Simulations and Measurement Lab – Michigan State University, Lansing June 4 -15, 2007
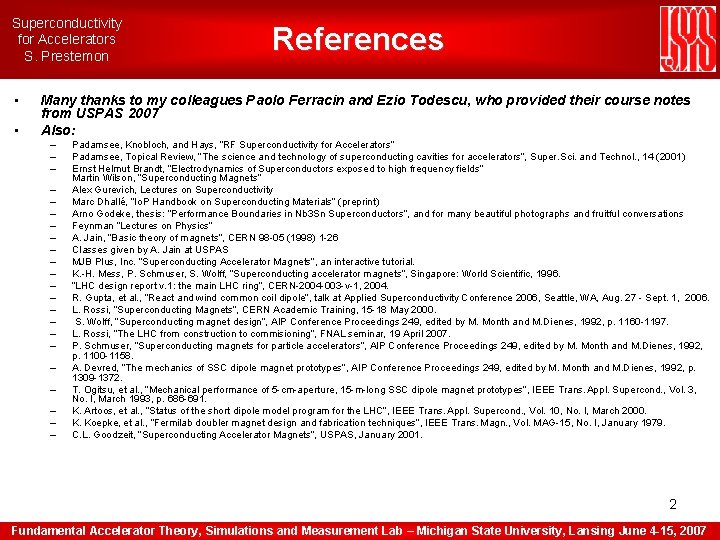
Superconductivity for Accelerators S. Prestemon • • References Many thanks to my colleagues Paolo Ferracin and Ezio Todescu, who provided their course notes from USPAS 2007 Also: – – – – – – Padamsee, Knobloch, and Hays, “RF Superconductivity for Accelerators” Padamsee, Topical Review, “The science and technology of superconducting cavities for accelerators”, Super. Sci. and Technol. , 14 (2001) Ernst Helmut Brandt, “Electrodynamics of Superconductors exposed to high frequency fields” Martin Wilson, “Superconducting Magnets” Alex Gurevich, Lectures on Superconductivity Marc Dhallé, “Io. P Handbook on Superconducting Materials” (preprint) Arno Godeke, thesis: “Performance Boundaries in Nb 3 Sn Superconductors”, and for many beautiful photographs and fruitful conversations Feynman “Lectures on Physics” A. Jain, “Basic theory of magnets”, CERN 98 -05 (1998) 1 -26 Classes given by A. Jain at USPAS MJB Plus, Inc. “Superconducting Accelerator Magnets”, an interactive tutorial. K. -H. Mess, P. Schmuser, S. Wolff, “Superconducting accelerator magnets”, Singapore: World Scientific, 1996. “LHC design report v. 1: the main LHC ring”, CERN-2004 -003 -v-1, 2004. R. Gupta, et al. , “React and wind common coil dipole”, talk at Applied Superconductivity Conference 2006, Seattle, WA, Aug. 27 - Sept. 1, 2006. L. Rossi, “Superconducting Magnets”, CERN Academic Training, 15 -18 May 2000. S. Wolff, “Superconducting magnet design”, AIP Conference Proceedings 249, edited by M. Month and M. Dienes, 1992, p. 1160 -1197. L. Rossi, “The LHC from construction to commisioning”, FNAL seminar, 19 April 2007. P. Schmuser, “Superconducting magnets for particle accelerators”, AIP Conference Proceedings 249, edited by M. Month and M. Dienes, 1992, p. 1100 -1158. A. Devred, “The mechanics of SSC dipole magnet prototypes”, AIP Conference Proceedings 249, edited by M. Month and M. Dienes, 1992, p. 1309 -1372. T. Ogitsu, et al. , “Mechanical performance of 5 -cm-aperture, 15 -m-long SSC dipole magnet prototypes”, IEEE Trans. Appl. Supercond. , Vol. 3, No. l, March 1993, p. 686 -691. K. Artoos, et al. , “Status of the short dipole model program for the LHC”, IEEE Trans. Appl. Supercond. , Vol. 10, No. l, March 2000. K. Koepke, et al. , “Fermilab doubler magnet design and fabrication techniques”, IEEE Trans. Magn. , Vol. MAG-15, No. l, January 1979. C. L. Goodzeit, “Superconducting Accelerator Magnets”, USPAS, January 2001. 2 Fundamental Accelerator Theory, Simulations and Measurement Lab – Michigan State University, Lansing June 4 -15, 2007
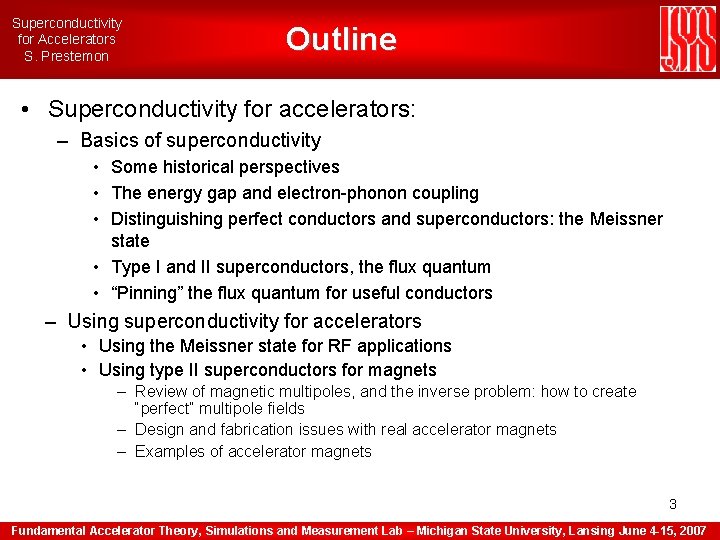
Superconductivity for Accelerators S. Prestemon Outline • Superconductivity for accelerators: – Basics of superconductivity • Some historical perspectives • The energy gap and electron-phonon coupling • Distinguishing perfect conductors and superconductors: the Meissner state • Type I and II superconductors, the flux quantum • “Pinning” the flux quantum for useful conductors – Using superconductivity for accelerators • Using the Meissner state for RF applications • Using type II superconductors for magnets – Review of magnetic multipoles, and the inverse problem: how to create “perfect” multipole fields – Design and fabrication issues with real accelerator magnets – Examples of accelerator magnets 3 Fundamental Accelerator Theory, Simulations and Measurement Lab – Michigan State University, Lansing June 4 -15, 2007
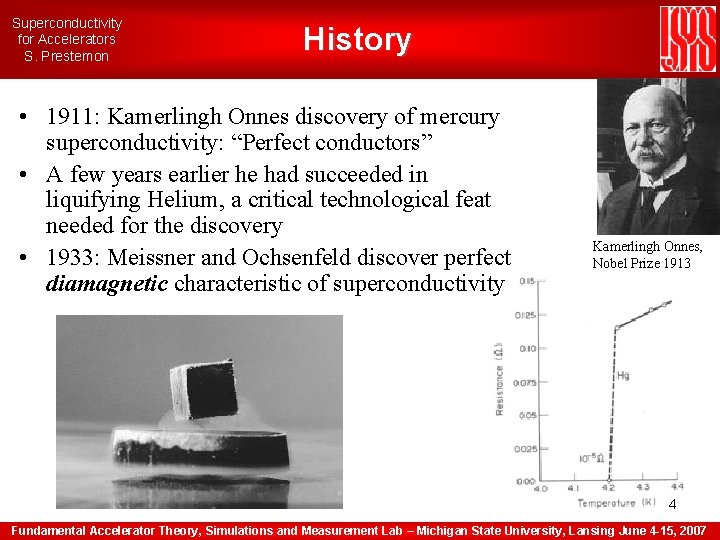
Superconductivity for Accelerators S. Prestemon History • 1911: Kamerlingh Onnes discovery of mercury superconductivity: “Perfect conductors” • A few years earlier he had succeeded in liquifying Helium, a critical technological feat needed for the discovery • 1933: Meissner and Ochsenfeld discover perfect diamagnetic characteristic of superconductivity Kamerlingh Onnes, Nobel Prize 1913 4 Fundamental Accelerator Theory, Simulations and Measurement Lab – Michigan State University, Lansing June 4 -15, 2007
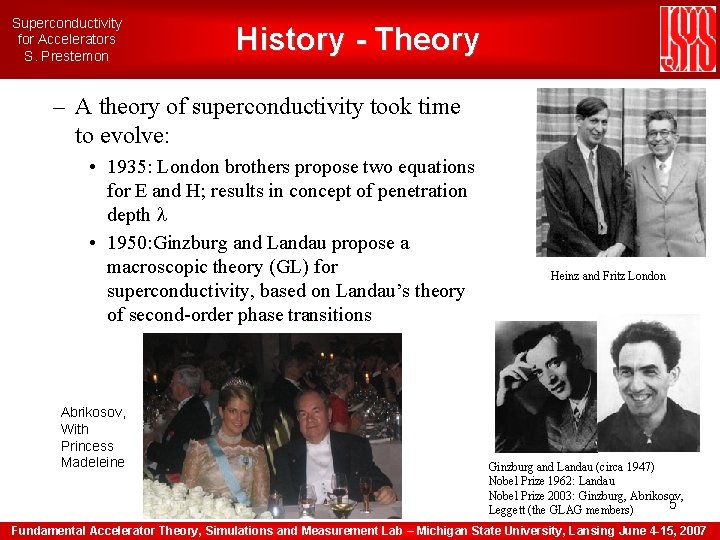
Superconductivity for Accelerators S. Prestemon History - Theory – A theory of superconductivity took time to evolve: • 1935: London brothers propose two equations for E and H; results in concept of penetration depth • 1950: Ginzburg and Landau propose a macroscopic theory (GL) for superconductivity, based on Landau’s theory of second-order phase transitions Abrikosov, With Princess Madeleine Heinz and Fritz London Ginzburg and Landau (circa 1947) Nobel Prize 1962: Landau Nobel Prize 2003: Ginzburg, Abrikosov, 5 Leggett (the GLAG members) Fundamental Accelerator Theory, Simulations and Measurement Lab – Michigan State University, Lansing June 4 -15, 2007
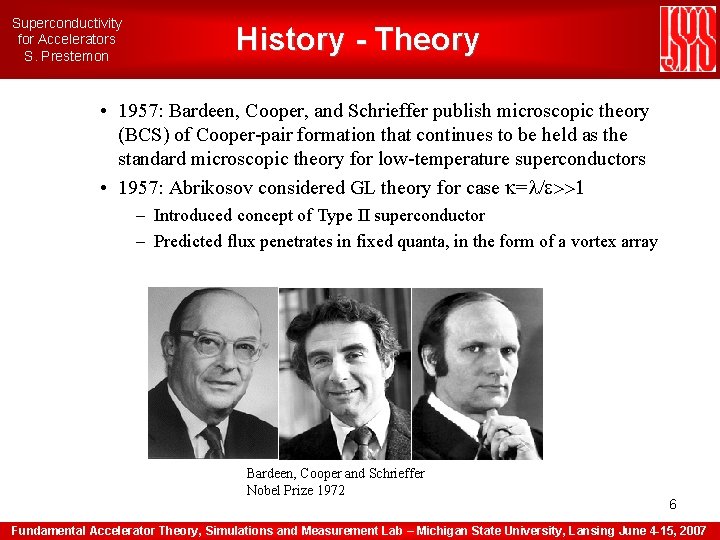
Superconductivity for Accelerators S. Prestemon History - Theory • 1957: Bardeen, Cooper, and Schrieffer publish microscopic theory (BCS) of Cooper-pair formation that continues to be held as the standard microscopic theory for low-temperature superconductors • 1957: Abrikosov considered GL theory for case = – Introduced concept of Type II superconductor – Predicted flux penetrates in fixed quanta, in the form of a vortex array Bardeen, Cooper and Schrieffer Nobel Prize 1972 6 Fundamental Accelerator Theory, Simulations and Measurement Lab – Michigan State University, Lansing June 4 -15, 2007
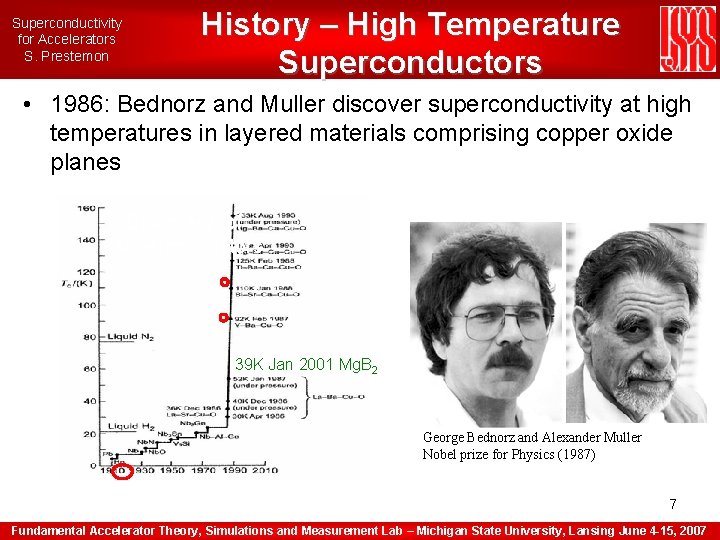
Superconductivity for Accelerators S. Prestemon History – High Temperature Superconductors • 1986: Bednorz and Muller discover superconductivity at high temperatures in layered materials comprising copper oxide planes Discovery of superconductors 39 K Jan 2001 Mg. B 2 George Bednorz and Alexander Muller Nobel prize for Physics (1987) 7 Fundamental Accelerator Theory, Simulations and Measurement Lab – Michigan State University, Lansing June 4 -15, 2007
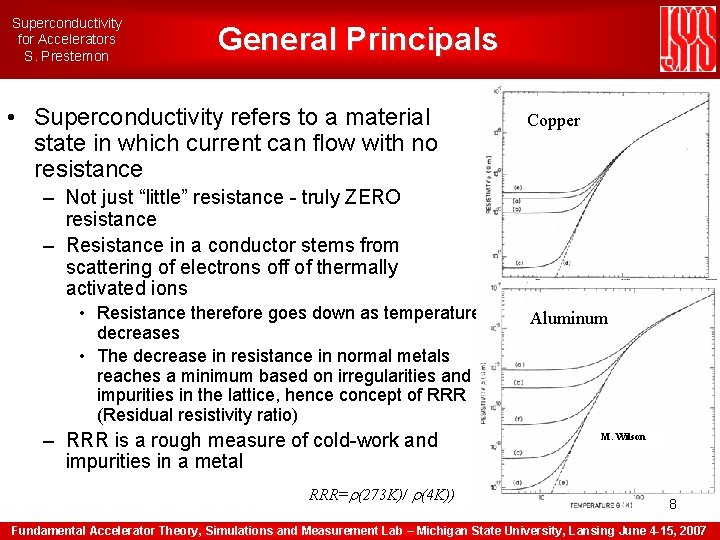
Superconductivity for Accelerators S. Prestemon General Principals • Superconductivity refers to a material state in which current can flow with no resistance Copper – Not just “little” resistance - truly ZERO resistance – Resistance in a conductor stems from scattering of electrons off of thermally activated ions • Resistance therefore goes down as temperature decreases • The decrease in resistance in normal metals reaches a minimum based on irregularities and impurities in the lattice, hence concept of RRR (Residual resistivity ratio) – RRR is a rough measure of cold-work and impurities in a metal RRR= (273 K)/ (4 K)) Aluminum M. Wilson 8 Fundamental Accelerator Theory, Simulations and Measurement Lab – Michigan State University, Lansing June 4 -15, 2007
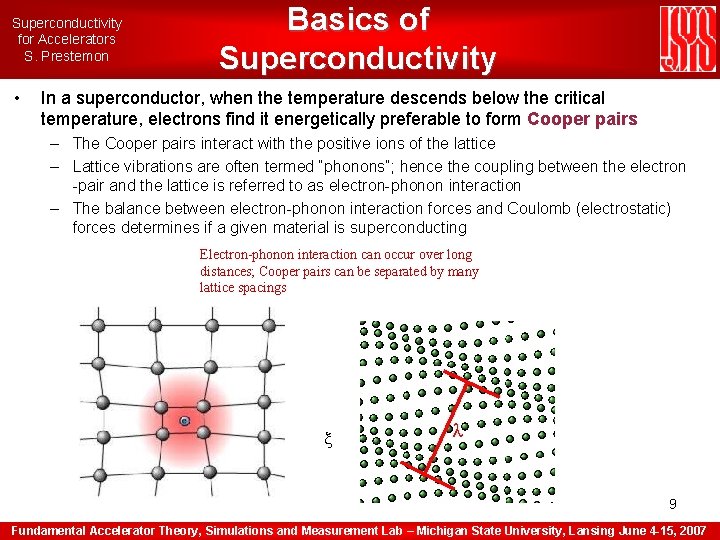
Superconductivity for Accelerators S. Prestemon • Basics of Superconductivity In a superconductor, when the temperature descends below the critical temperature, electrons find it energetically preferable to form Cooper pairs – The Cooper pairs interact with the positive ions of the lattice – Lattice vibrations are often termed “phonons”; hence the coupling between the electron -pair and the lattice is referred to as electron-phonon interaction – The balance between electron-phonon interaction forces and Coulomb (electrostatic) forces determines if a given material is superconducting Electron-phonon interaction can occur over long distances; Cooper pairs can be separated by many lattice spacings x 9 Fundamental Accelerator Theory, Simulations and Measurement Lab – Michigan State University, Lansing June 4 -15, 2007
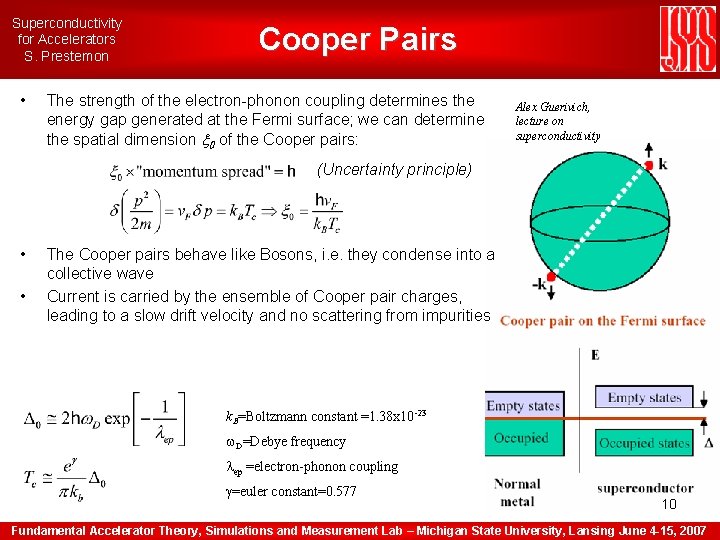
Superconductivity for Accelerators S. Prestemon • Cooper Pairs The strength of the electron-phonon coupling determines the energy gap generated at the Fermi surface; we can determine the spatial dimension x 0 of the Cooper pairs: Alex Guerivich, lecture on superconductivity (Uncertainty principle) • • The Cooper pairs behave like Bosons, i. e. they condense into a collective wave Current is carried by the ensemble of Cooper pair charges, leading to a slow drift velocity and no scattering from impurities k. B=Boltzmann constant =1. 38 x 10 -23 D=Debye frequency ep =electron-phonon coupling g=euler constant=0. 577 10 Fundamental Accelerator Theory, Simulations and Measurement Lab – Michigan State University, Lansing June 4 -15, 2007
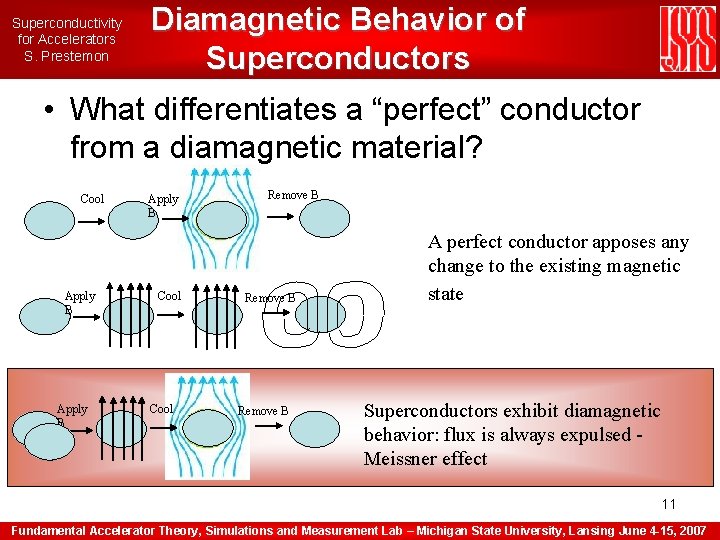
Superconductivity for Accelerators S. Prestemon Diamagnetic Behavior of Superconductors • What differentiates a “perfect” conductor from a diamagnetic material? Cool Apply B Cool Remove B A perfect conductor apposes any change to the existing magnetic state Superconductors exhibit diamagnetic behavior: flux is always expulsed Meissner effect 11 Fundamental Accelerator Theory, Simulations and Measurement Lab – Michigan State University, Lansing June 4 -15, 2007
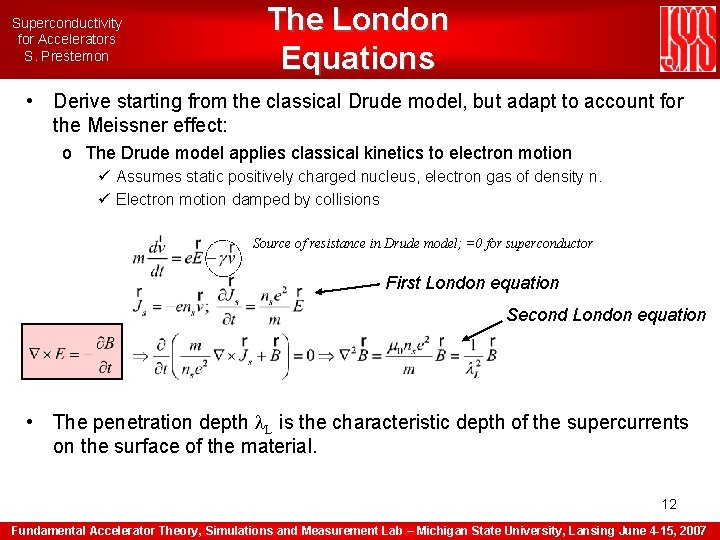
Superconductivity for Accelerators S. Prestemon The London Equations • Derive starting from the classical Drude model, but adapt to account for the Meissner effect: o The Drude model applies classical kinetics to electron motion ü Assumes static positively charged nucleus, electron gas of density n. ü Electron motion damped by collisions Source of resistance in Drude model; =0 for superconductor First London equation Second London equation • The penetration depth L is the characteristic depth of the supercurrents on the surface of the material. 12 Fundamental Accelerator Theory, Simulations and Measurement Lab – Michigan State University, Lansing June 4 -15, 2007
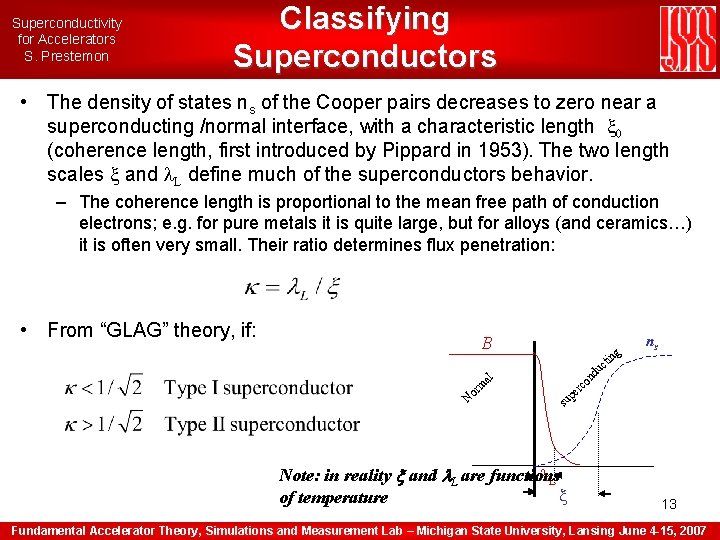
Superconductivity for Accelerators S. Prestemon Classifying Superconductors • The density of states ns of the Cooper pairs decreases to zero near a superconducting /normal interface, with a characteristic length x 0 (coherence length, first introduced by Pippard in 1953). The two length scales x and L define much of the superconductors behavior. – The coherence length is proportional to the mean free path of conduction electrons; e. g. for pure metals it is quite large, but for alloys (and ceramics…) it is often very small. Their ratio determines flux penetration: • From “GLAG” theory, if: B al o rm N n co r pe c du ng ns ti su L Note: in reality x and l. L are functions x of temperature 13 Fundamental Accelerator Theory, Simulations and Measurement Lab – Michigan State University, Lansing June 4 -15, 2007
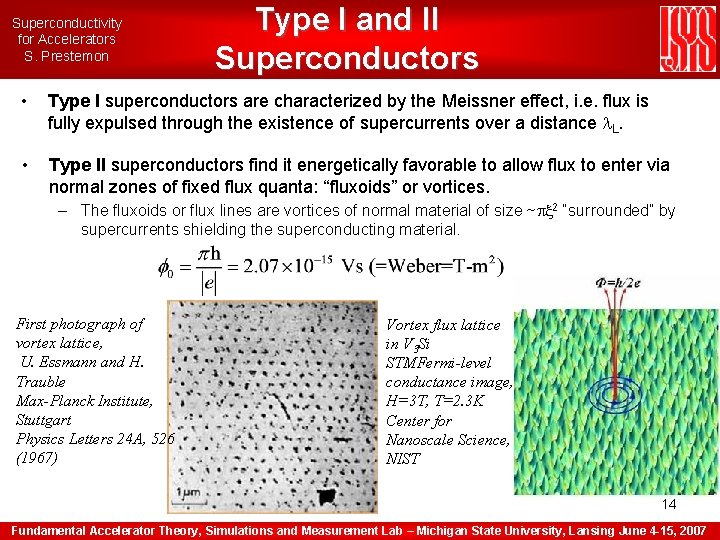
Superconductivity for Accelerators S. Prestemon Type I and II Superconductors • Type I superconductors are characterized by the Meissner effect, i. e. flux is fully expulsed through the existence of supercurrents over a distance L. • Type II superconductors find it energetically favorable to allow flux to enter via normal zones of fixed flux quanta: “fluxoids” or vortices. – The fluxoids or flux lines are vortices of normal material of size ~ x 2 “surrounded” by supercurrents shielding the superconducting material. First photograph of vortex lattice, U. Essmann and H. Trauble Max-Planck Institute, Stuttgart Physics Letters 24 A, 526 (1967) Vortex flux lattice in V 3 Si STMFermi-level conductance image, H=3 T, T=2. 3 K Center for Nanoscale Science, NIST 14 Fundamental Accelerator Theory, Simulations and Measurement Lab – Michigan State University, Lansing June 4 -15, 2007
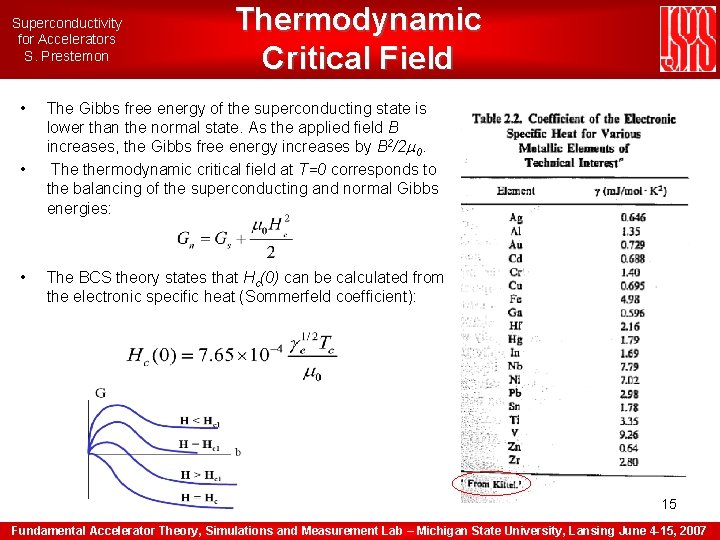
Superconductivity for Accelerators S. Prestemon • • • Thermodynamic Critical Field The Gibbs free energy of the superconducting state is lower than the normal state. As the applied field B increases, the Gibbs free energy increases by B 2/2 m 0. The thermodynamic critical field at T=0 corresponds to the balancing of the superconducting and normal Gibbs energies: The BCS theory states that Hc(0) can be calculated from the electronic specific heat (Sommerfeld coefficient): 15 Fundamental Accelerator Theory, Simulations and Measurement Lab – Michigan State University, Lansing June 4 -15, 2007
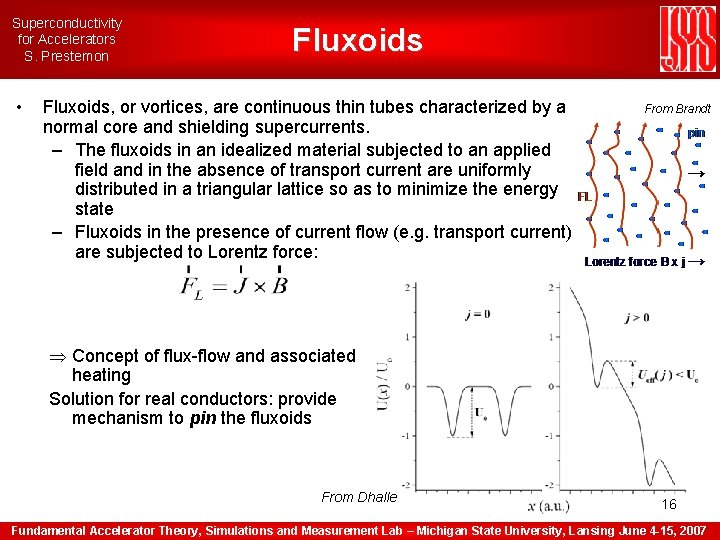
Superconductivity for Accelerators S. Prestemon • Fluxoids, or vortices, are continuous thin tubes characterized by a normal core and shielding supercurrents. – The fluxoids in an idealized material subjected to an applied field and in the absence of transport current are uniformly distributed in a triangular lattice so as to minimize the energy state – Fluxoids in the presence of current flow (e. g. transport current) are subjected to Lorentz force: From Brandt Þ Concept of flux-flow and associated heating Solution for real conductors: provide mechanism to pin the fluxoids From Dhalle 16 Fundamental Accelerator Theory, Simulations and Measurement Lab – Michigan State University, Lansing June 4 -15, 2007
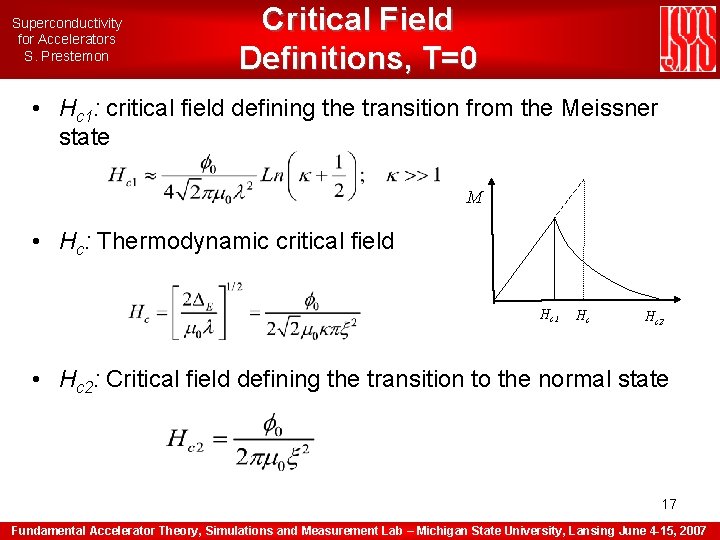
Superconductivity for Accelerators S. Prestemon Critical Field Definitions, T=0 • Hc 1: critical field defining the transition from the Meissner state M • Hc: Thermodynamic critical field Hc 1 Hc Hc 2 • Hc 2: Critical field defining the transition to the normal state 17 Fundamental Accelerator Theory, Simulations and Measurement Lab – Michigan State University, Lansing June 4 -15, 2007
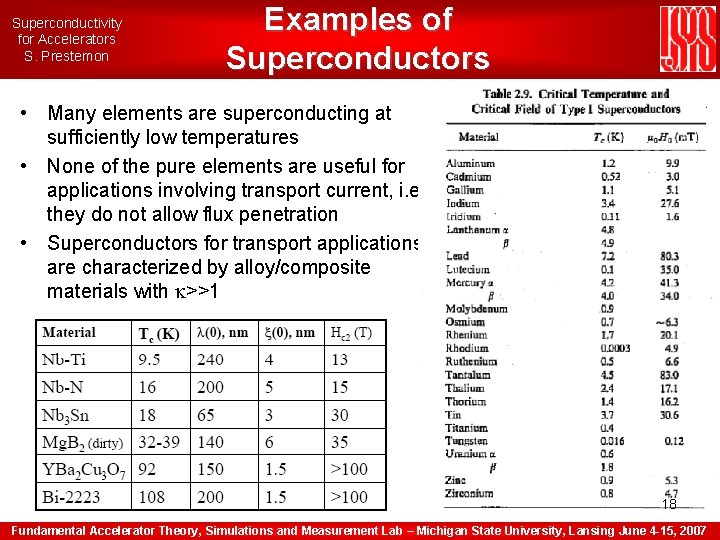
Superconductivity for Accelerators S. Prestemon Examples of Superconductors • Many elements are superconducting at sufficiently low temperatures • None of the pure elements are useful for applications involving transport current, i. e. they do not allow flux penetration • Superconductors for transport applications are characterized by alloy/composite materials with >>1 18 Fundamental Accelerator Theory, Simulations and Measurement Lab – Michigan State University, Lansing June 4 -15, 2007
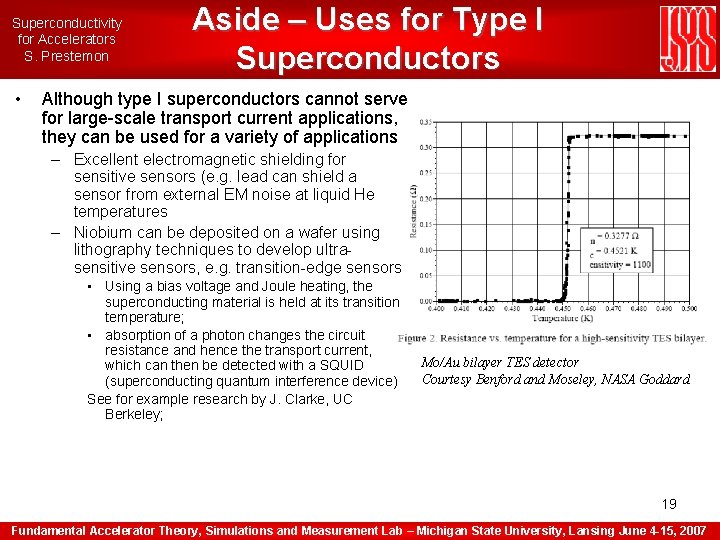
Superconductivity for Accelerators S. Prestemon • Aside – Uses for Type I Superconductors Although type I superconductors cannot serve for large-scale transport current applications, they can be used for a variety of applications – Excellent electromagnetic shielding for sensitive sensors (e. g. lead can shield a sensor from external EM noise at liquid He temperatures – Niobium can be deposited on a wafer using lithography techniques to develop ultrasensitive sensors, e. g. transition-edge sensors • Using a bias voltage and Joule heating, the superconducting material is held at its transition temperature; • absorption of a photon changes the circuit resistance and hence the transport current, which can then be detected with a SQUID (superconducting quantum interference device) See for example research by J. Clarke, UC Berkeley; Mo/Au bilayer TES detector Courtesy Benford and Moseley, NASA Goddard 19 Fundamental Accelerator Theory, Simulations and Measurement Lab – Michigan State University, Lansing June 4 -15, 2007
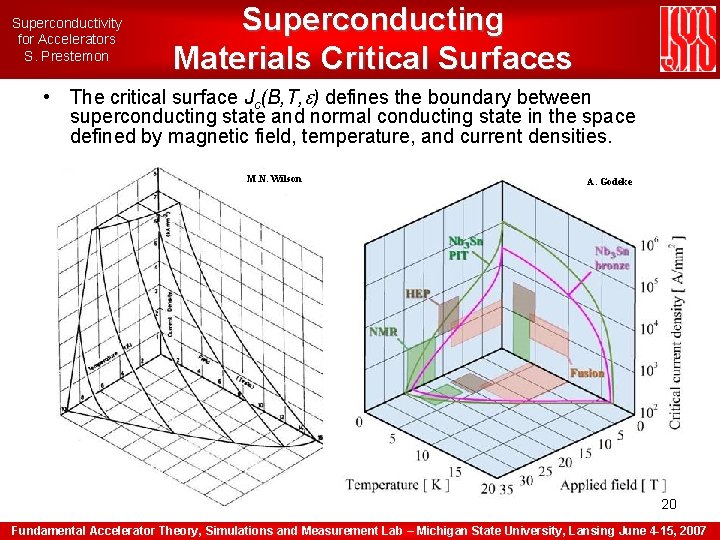
Superconductivity for Accelerators S. Prestemon Superconducting Materials Critical Surfaces • The critical surface Jc(B, T, e) defines the boundary between superconducting state and normal conducting state in the space defined by magnetic field, temperature, and current densities. M. N. Wilson A. Godeke 20 Fundamental Accelerator Theory, Simulations and Measurement Lab – Michigan State University, Lansing June 4 -15, 2007
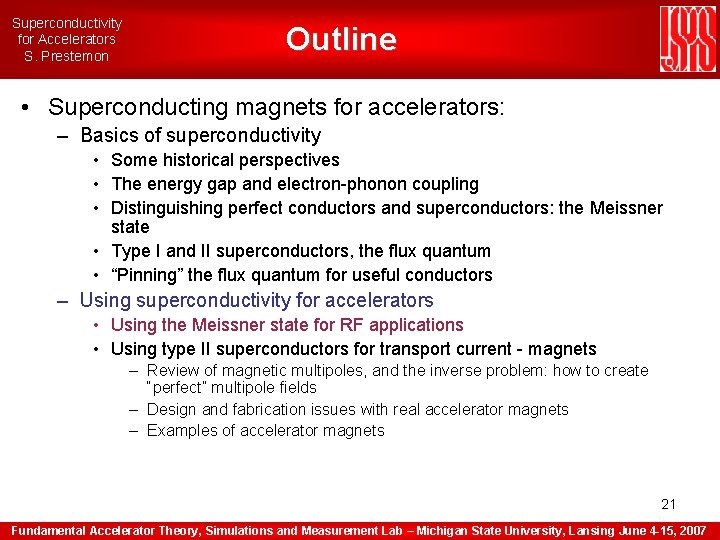
Superconductivity for Accelerators S. Prestemon Outline • Superconducting magnets for accelerators: – Basics of superconductivity • Some historical perspectives • The energy gap and electron-phonon coupling • Distinguishing perfect conductors and superconductors: the Meissner state • Type I and II superconductors, the flux quantum • “Pinning” the flux quantum for useful conductors – Using superconductivity for accelerators • Using the Meissner state for RF applications • Using type II superconductors for transport current - magnets – Review of magnetic multipoles, and the inverse problem: how to create “perfect” multipole fields – Design and fabrication issues with real accelerator magnets – Examples of accelerator magnets 21 Fundamental Accelerator Theory, Simulations and Measurement Lab – Michigan State University, Lansing June 4 -15, 2007
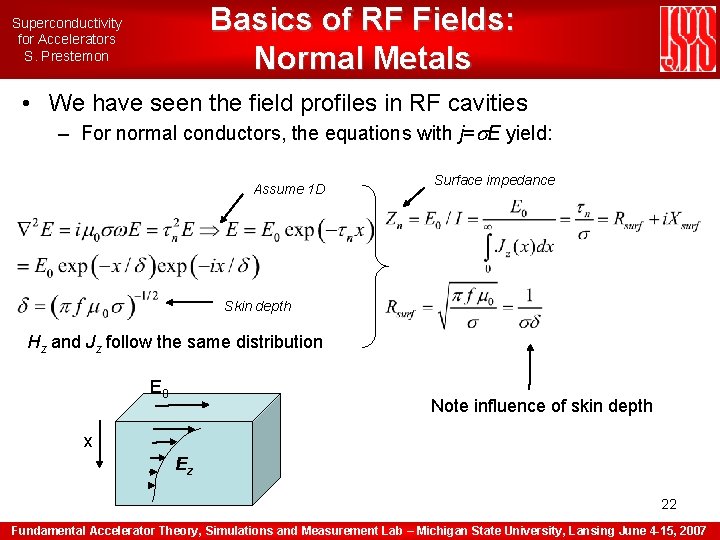
Basics of RF Fields: Normal Metals Superconductivity for Accelerators S. Prestemon • We have seen the field profiles in RF cavities – For normal conductors, the equations with j=s. E yield: Assume 1 D Surface impedance Skin depth Hz and Jz follow the same distribution E 0 Note influence of skin depth x Ez 22 Fundamental Accelerator Theory, Simulations and Measurement Lab – Michigan State University, Lansing June 4 -15, 2007
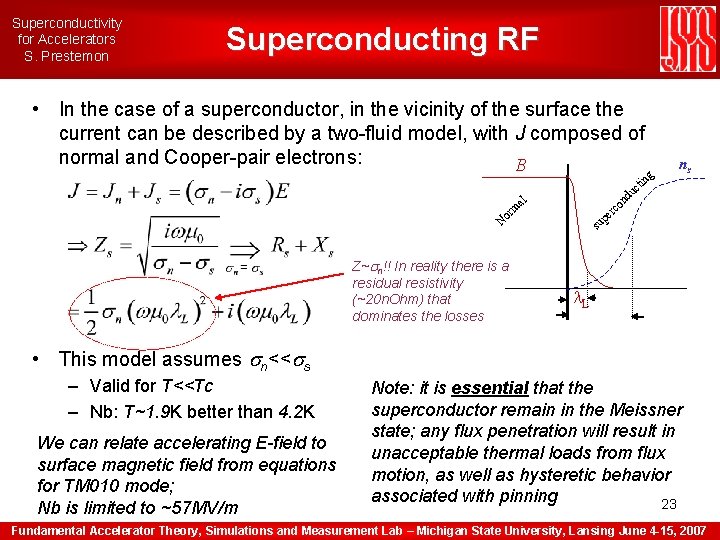
Superconductivity for Accelerators S. Prestemon Superconducting RF • In the case of a superconductor, in the vicinity of the surface the current can be described by a two-fluid model, with J composed of normal and Cooper-pair electrons: B c al rm N Z~sn!! In reality there is a residual resistivity (~20 n. Ohm) that dominates the losses ns o rc e up o u nd g tin s L • This model assumes sn<<ss – Valid for T<<Tc – Nb: T~1. 9 K better than 4. 2 K We can relate accelerating E-field to surface magnetic field from equations for TM 010 mode; Nb is limited to ~57 MV/m Note: it is essential that the superconductor remain in the Meissner state; any flux penetration will result in unacceptable thermal loads from flux motion, as well as hysteretic behavior associated with pinning 23 Fundamental Accelerator Theory, Simulations and Measurement Lab – Michigan State University, Lansing June 4 -15, 2007
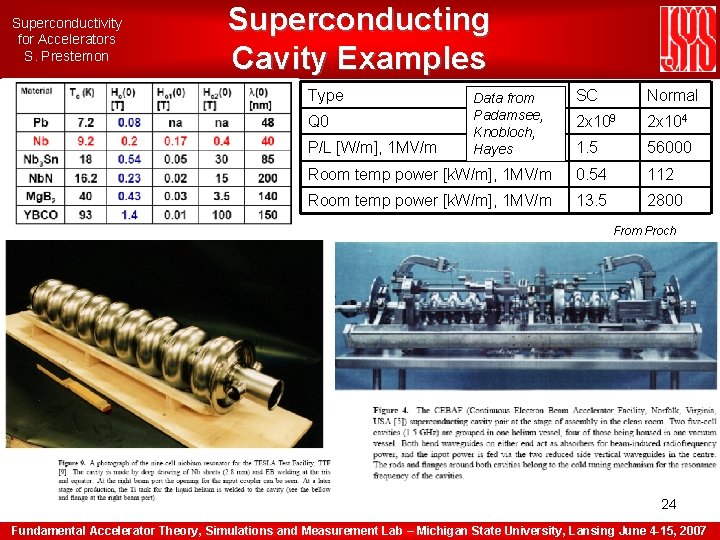
Superconductivity for Accelerators S. Prestemon Superconducting Cavity Examples Type SC Normal 2 x 109 2 x 104 1. 5 56000 Room temp power [k. W/m], 1 MV/m 0. 54 112 Room temp power [k. W/m], 1 MV/m 13. 5 2800 Q 0 P/L [W/m], 1 MV/m Data from Padamsee, Knobloch, Hayes From Proch 24 Fundamental Accelerator Theory, Simulations and Measurement Lab – Michigan State University, Lansing June 4 -15, 2007
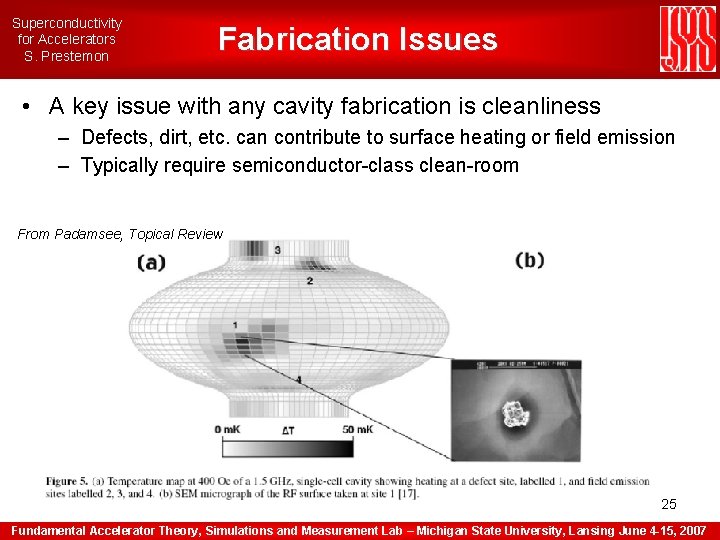
Superconductivity for Accelerators S. Prestemon Fabrication Issues • A key issue with any cavity fabrication is cleanliness – Defects, dirt, etc. can contribute to surface heating or field emission – Typically require semiconductor-class clean-room From Padamsee, Topical Review 25 Fundamental Accelerator Theory, Simulations and Measurement Lab – Michigan State University, Lansing June 4 -15, 2007
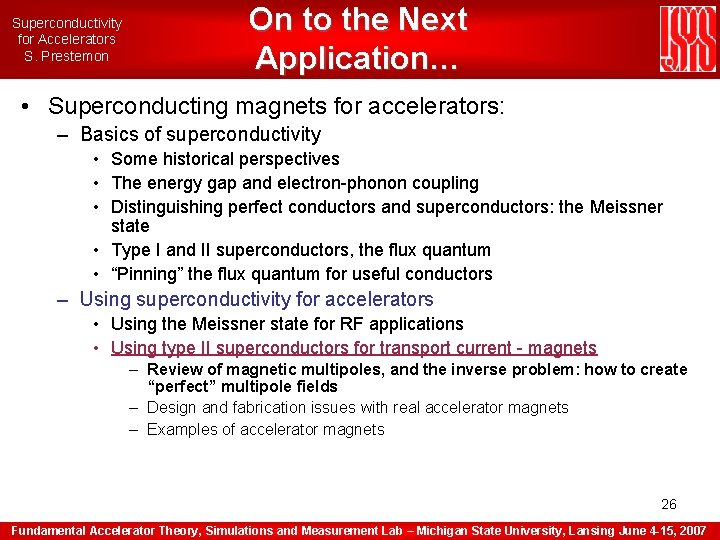
Superconductivity for Accelerators S. Prestemon On to the Next Application… • Superconducting magnets for accelerators: – Basics of superconductivity • Some historical perspectives • The energy gap and electron-phonon coupling • Distinguishing perfect conductors and superconductors: the Meissner state • Type I and II superconductors, the flux quantum • “Pinning” the flux quantum for useful conductors – Using superconductivity for accelerators • Using the Meissner state for RF applications • Using type II superconductors for transport current - magnets – Review of magnetic multipoles, and the inverse problem: how to create “perfect” multipole fields – Design and fabrication issues with real accelerator magnets – Examples of accelerator magnets 26 Fundamental Accelerator Theory, Simulations and Measurement Lab – Michigan State University, Lansing June 4 -15, 2007
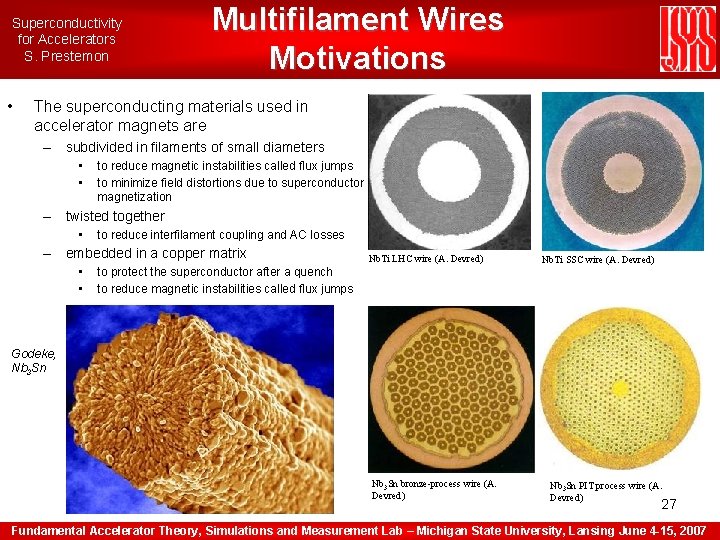
Superconductivity for Accelerators S. Prestemon • Multifilament Wires Motivations The superconducting materials used in accelerator magnets are – subdivided in filaments of small diameters • • to reduce magnetic instabilities called flux jumps to minimize field distortions due to superconductor magnetization – twisted together • to reduce interfilament coupling and AC losses – embedded in a copper matrix • • Nb. Ti LHC wire (A. Devred) Nb. Ti SSC wire (A. Devred) to protect the superconductor after a quench to reduce magnetic instabilities called flux jumps Godeke, Nb 3 Sn bronze-process wire (A. Devred) Nb 3 Sn PIT process wire (A. Devred) 27 Fundamental Accelerator Theory, Simulations and Measurement Lab – Michigan State University, Lansing June 4 -15, 2007
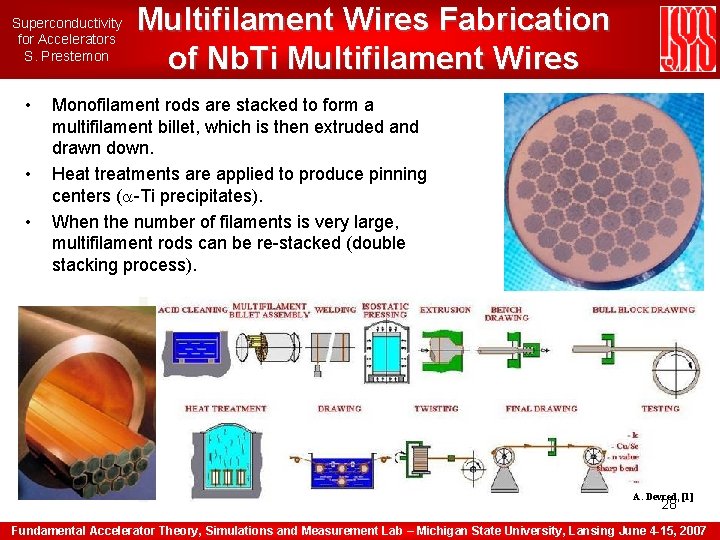
Superconductivity for Accelerators S. Prestemon • • • Multifilament Wires Fabrication of Nb. Ti Multifilament Wires Monofilament rods are stacked to form a multifilament billet, which is then extruded and drawn down. Heat treatments are applied to produce pinning centers ( -Ti precipitates). When the number of filaments is very large, multifilament rods can be re-stacked (double stacking process). A. Devred, [1] 28 Fundamental Accelerator Theory, Simulations and Measurement Lab – Michigan State University, Lansing June 4 -15, 2007
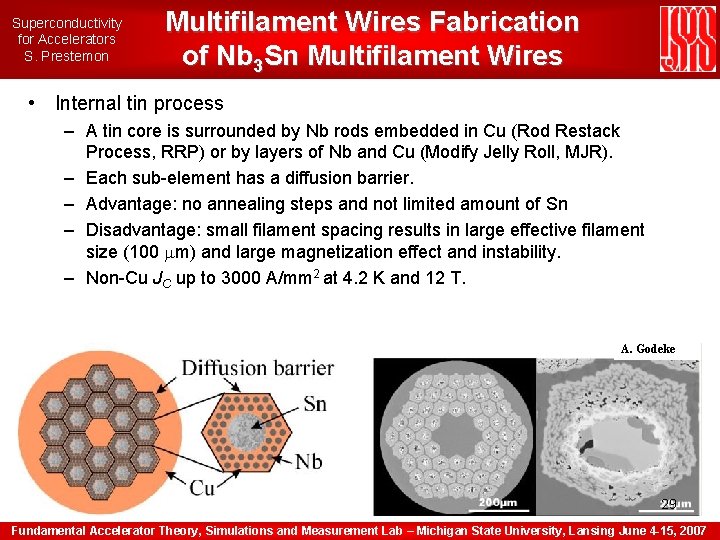
Superconductivity for Accelerators S. Prestemon Multifilament Wires Fabrication of Nb 3 Sn Multifilament Wires • Internal tin process – A tin core is surrounded by Nb rods embedded in Cu (Rod Restack Process, RRP) or by layers of Nb and Cu (Modify Jelly Roll, MJR). – Each sub-element has a diffusion barrier. – Advantage: no annealing steps and not limited amount of Sn – Disadvantage: small filament spacing results in large effective filament size (100 m) and large magnetization effect and instability. – Non-Cu JC up to 3000 A/mm 2 at 4. 2 K and 12 T. A. Godeke 29 Fundamental Accelerator Theory, Simulations and Measurement Lab – Michigan State University, Lansing June 4 -15, 2007
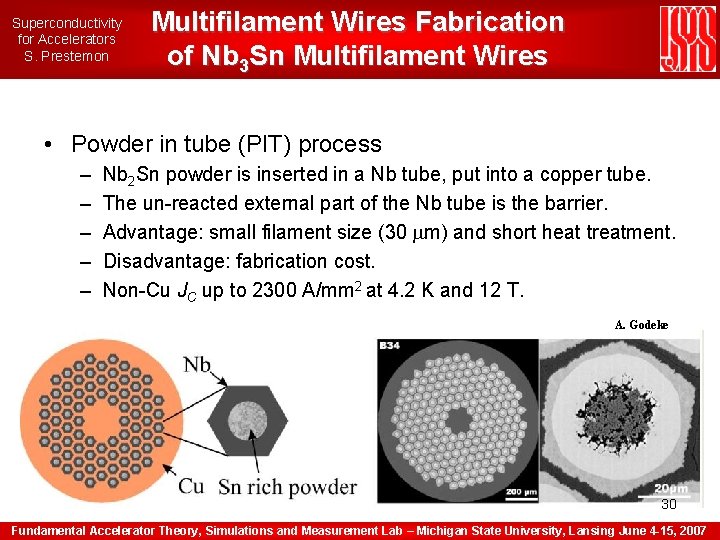
Superconductivity for Accelerators S. Prestemon Multifilament Wires Fabrication of Nb 3 Sn Multifilament Wires • Powder in tube (PIT) process – – – Nb 2 Sn powder is inserted in a Nb tube, put into a copper tube. The un-reacted external part of the Nb tube is the barrier. Advantage: small filament size (30 m) and short heat treatment. Disadvantage: fabrication cost. Non-Cu JC up to 2300 A/mm 2 at 4. 2 K and 12 T. A. Godeke 30 Fundamental Accelerator Theory, Simulations and Measurement Lab – Michigan State University, Lansing June 4 -15, 2007
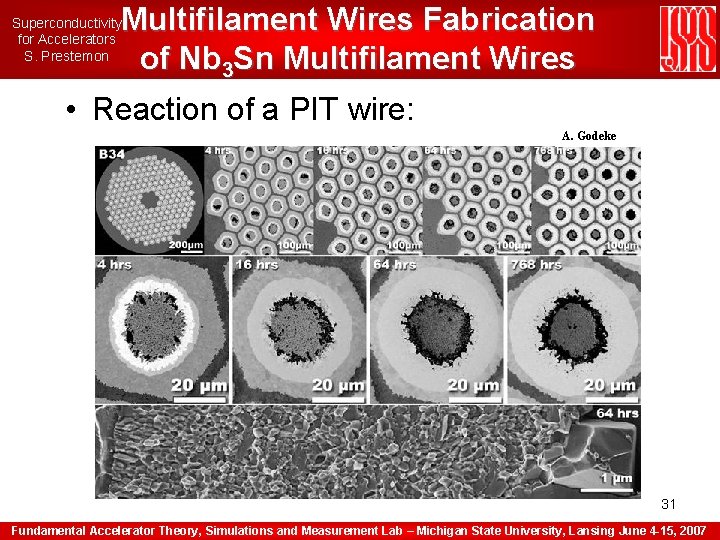
Multifilament Wires Fabrication of Nb 3 Sn Multifilament Wires Superconductivity for Accelerators S. Prestemon • Reaction of a PIT wire: A. Godeke 31 Fundamental Accelerator Theory, Simulations and Measurement Lab – Michigan State University, Lansing June 4 -15, 2007
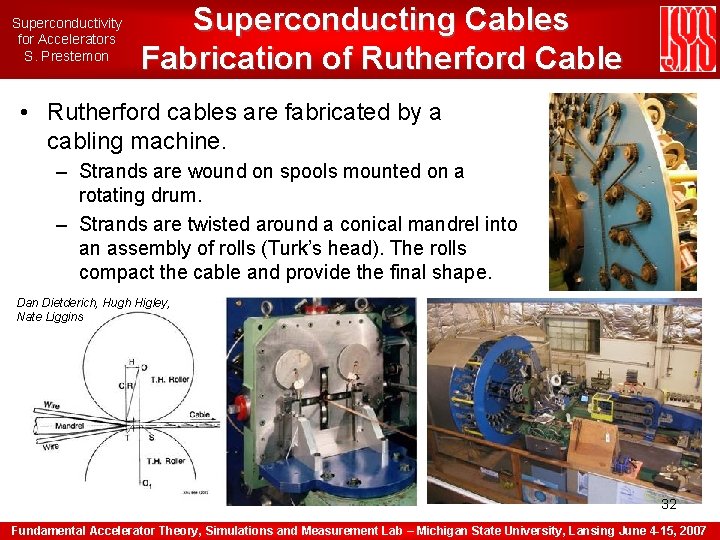
Superconductivity for Accelerators S. Prestemon Superconducting Cables Fabrication of Rutherford Cable • Rutherford cables are fabricated by a cabling machine. – Strands are wound on spools mounted on a rotating drum. – Strands are twisted around a conical mandrel into an assembly of rolls (Turk’s head). The rolls compact the cable and provide the final shape. Dan Dietderich, Hugh Higley, Nate Liggins 32 Fundamental Accelerator Theory, Simulations and Measurement Lab – Michigan State University, Lansing June 4 -15, 2007
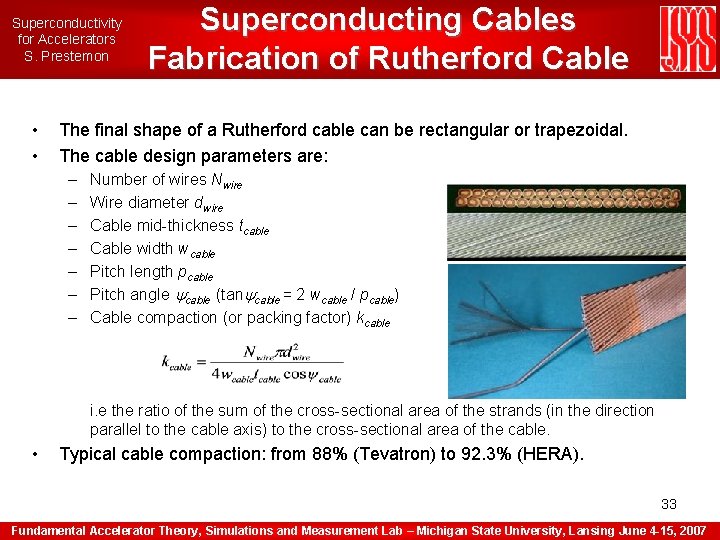
Superconductivity for Accelerators S. Prestemon • • Superconducting Cables Fabrication of Rutherford Cable The final shape of a Rutherford cable can be rectangular or trapezoidal. The cable design parameters are: – – – – Number of wires Nwire Wire diameter dwire Cable mid-thickness tcable Cable width wcable Pitch length pcable Pitch angle cable (tan cable = 2 wcable / pcable) Cable compaction (or packing factor) kcable i. e the ratio of the sum of the cross-sectional area of the strands (in the direction parallel to the cable axis) to the cross-sectional area of the cable. • Typical cable compaction: from 88% (Tevatron) to 92. 3% (HERA). 33 Fundamental Accelerator Theory, Simulations and Measurement Lab – Michigan State University, Lansing June 4 -15, 2007
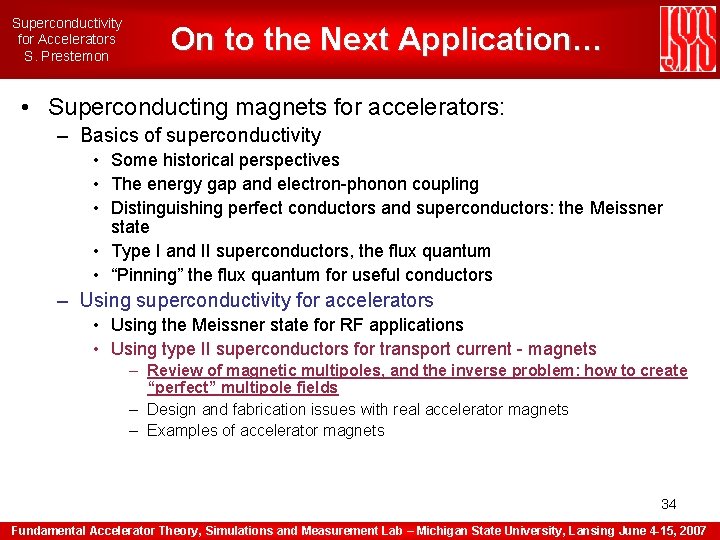
Superconductivity for Accelerators S. Prestemon On to the Next Application… • Superconducting magnets for accelerators: – Basics of superconductivity • Some historical perspectives • The energy gap and electron-phonon coupling • Distinguishing perfect conductors and superconductors: the Meissner state • Type I and II superconductors, the flux quantum • “Pinning” the flux quantum for useful conductors – Using superconductivity for accelerators • Using the Meissner state for RF applications • Using type II superconductors for transport current - magnets – Review of magnetic multipoles, and the inverse problem: how to create “perfect” multipole fields – Design and fabrication issues with real accelerator magnets – Examples of accelerator magnets 34 Fundamental Accelerator Theory, Simulations and Measurement Lab – Michigan State University, Lansing June 4 -15, 2007
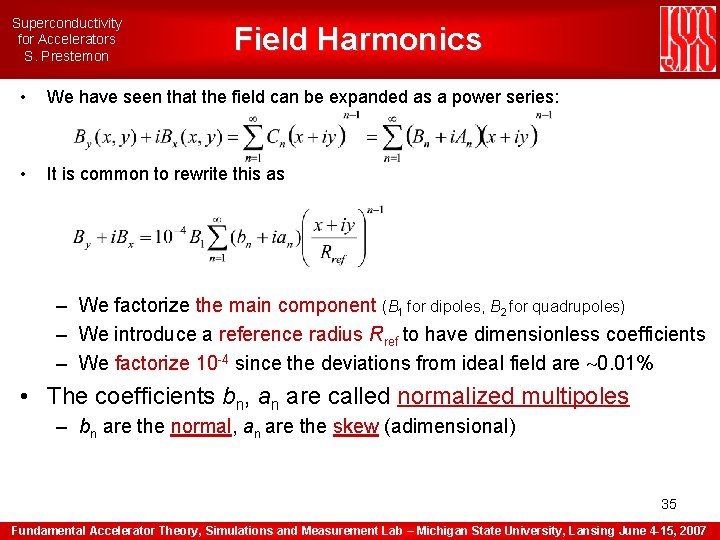
Superconductivity for Accelerators S. Prestemon Field Harmonics • We have seen that the field can be expanded as a power series: • It is common to rewrite this as – We factorize the main component (B 1 for dipoles, B 2 for quadrupoles) – We introduce a reference radius Rref to have dimensionless coefficients – We factorize 10 -4 since the deviations from ideal field are 0. 01% • The coefficients bn, an are called normalized multipoles – bn are the normal, an are the skew (adimensional) 35 Fundamental Accelerator Theory, Simulations and Measurement Lab – Michigan State University, Lansing June 4 -15, 2007
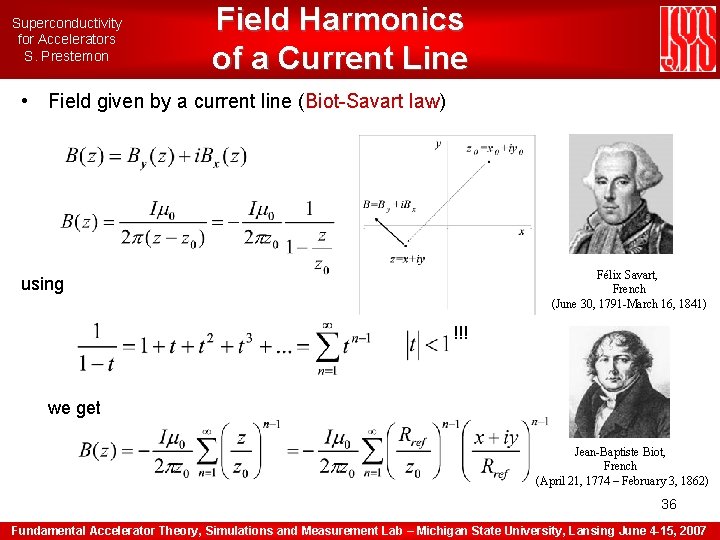
Superconductivity for Accelerators S. Prestemon Field Harmonics of a Current Line • Field given by a current line (Biot-Savart law) Félix Savart, French (June 30, 1791 -March 16, 1841) using !!! we get Jean-Baptiste Biot, French (April 21, 1774 – February 3, 1862) 36 Fundamental Accelerator Theory, Simulations and Measurement Lab – Michigan State University, Lansing June 4 -15, 2007
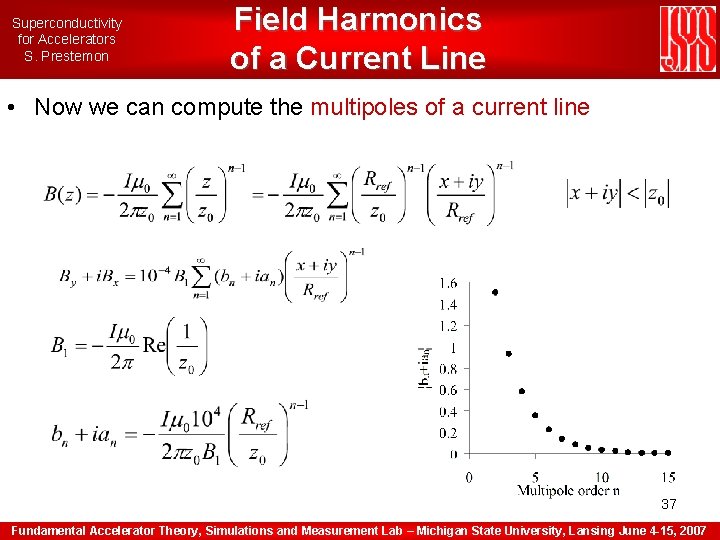
Superconductivity for Accelerators S. Prestemon Field Harmonics of a Current Line • Now we can compute the multipoles of a current line 37 Fundamental Accelerator Theory, Simulations and Measurement Lab – Michigan State University, Lansing June 4 -15, 2007
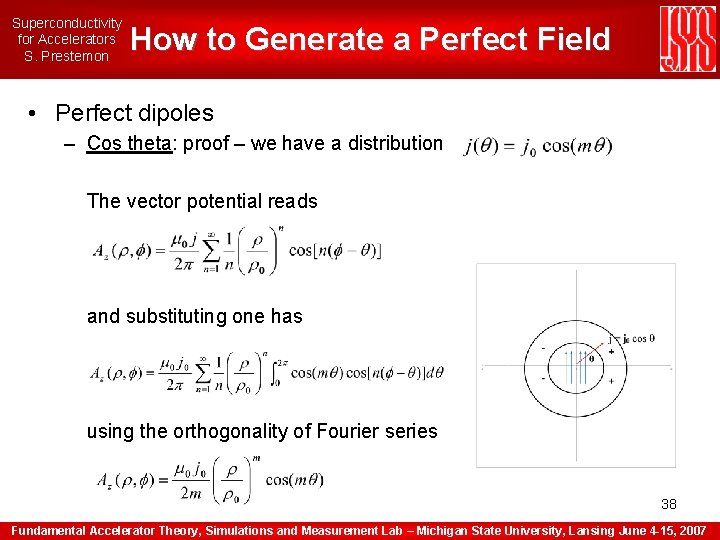
Superconductivity for Accelerators S. Prestemon How to Generate a Perfect Field • Perfect dipoles – Cos theta: proof – we have a distribution The vector potential reads and substituting one has using the orthogonality of Fourier series 38 Fundamental Accelerator Theory, Simulations and Measurement Lab – Michigan State University, Lansing June 4 -15, 2007
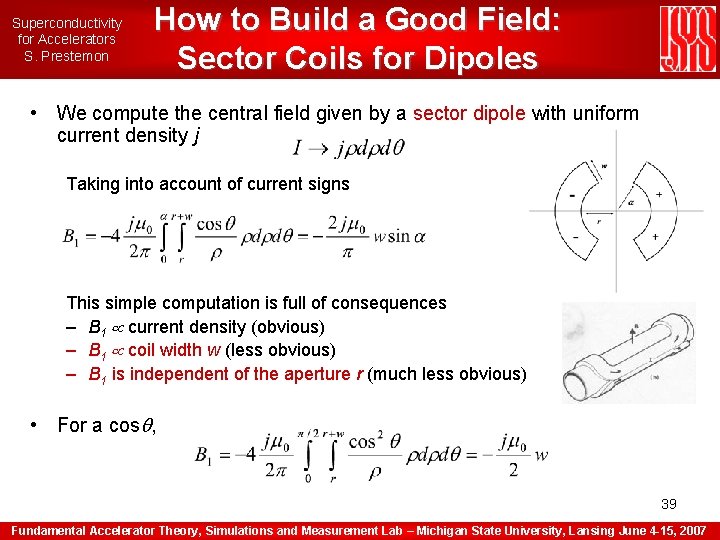
Superconductivity for Accelerators S. Prestemon How to Build a Good Field: Sector Coils for Dipoles • We compute the central field given by a sector dipole with uniform current density j Taking into account of current signs This simple computation is full of consequences – B 1 current density (obvious) – B 1 coil width w (less obvious) – B 1 is independent of the aperture r (much less obvious) • For a cos , 39 Fundamental Accelerator Theory, Simulations and Measurement Lab – Michigan State University, Lansing June 4 -15, 2007
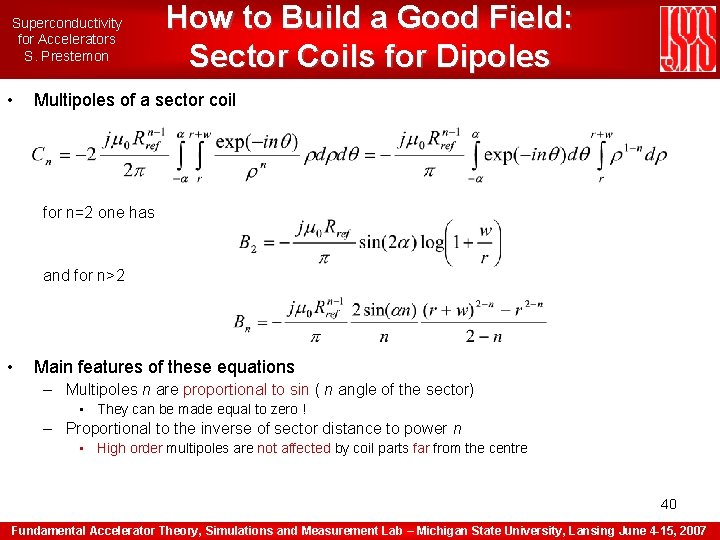
Superconductivity for Accelerators S. Prestemon • How to Build a Good Field: Sector Coils for Dipoles Multipoles of a sector coil for n=2 one has and for n>2 • Main features of these equations – Multipoles n are proportional to sin ( n angle of the sector) • They can be made equal to zero ! – Proportional to the inverse of sector distance to power n • High order multipoles are not affected by coil parts far from the centre 40 Fundamental Accelerator Theory, Simulations and Measurement Lab – Michigan State University, Lansing June 4 -15, 2007
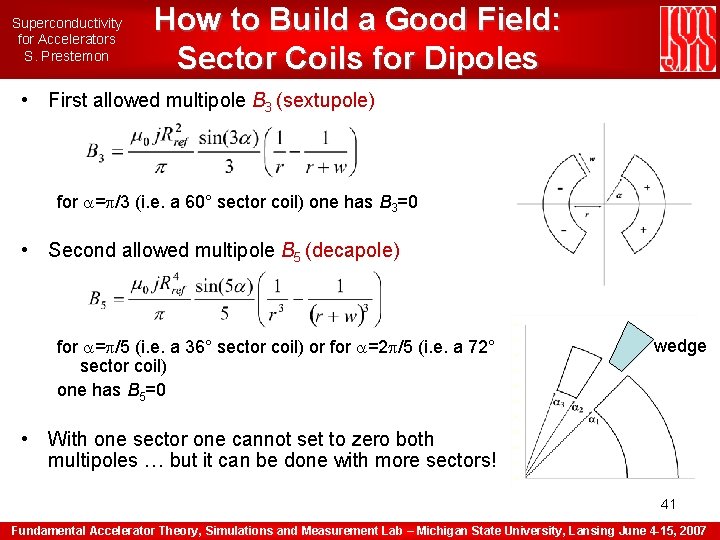
Superconductivity for Accelerators S. Prestemon How to Build a Good Field: Sector Coils for Dipoles • First allowed multipole B 3 (sextupole) for = /3 (i. e. a 60° sector coil) one has B 3=0 • Second allowed multipole B 5 (decapole) for = /5 (i. e. a 36° sector coil) or for =2 /5 (i. e. a 72° sector coil) one has B 5=0 wedge • With one sector one cannot set to zero both multipoles … but it can be done with more sectors! 41 Fundamental Accelerator Theory, Simulations and Measurement Lab – Michigan State University, Lansing June 4 -15, 2007
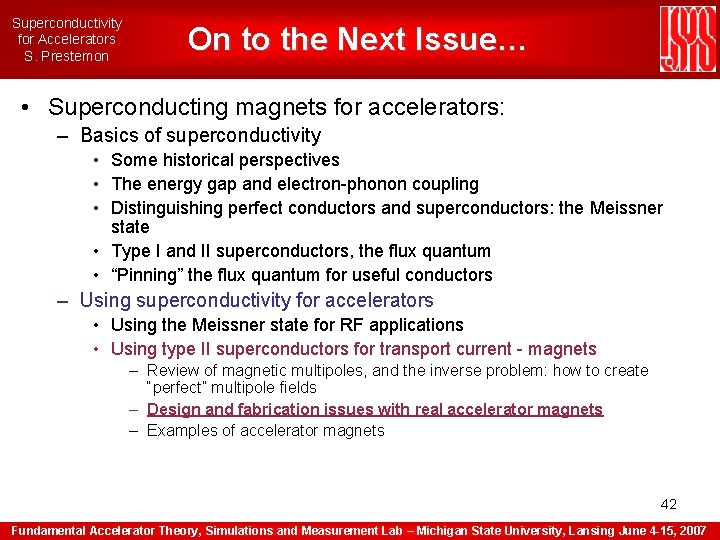
Superconductivity for Accelerators S. Prestemon On to the Next Issue… • Superconducting magnets for accelerators: – Basics of superconductivity • Some historical perspectives • The energy gap and electron-phonon coupling • Distinguishing perfect conductors and superconductors: the Meissner state • Type I and II superconductors, the flux quantum • “Pinning” the flux quantum for useful conductors – Using superconductivity for accelerators • Using the Meissner state for RF applications • Using type II superconductors for transport current - magnets – Review of magnetic multipoles, and the inverse problem: how to create “perfect” multipole fields – Design and fabrication issues with real accelerator magnets – Examples of accelerator magnets 42 Fundamental Accelerator Theory, Simulations and Measurement Lab – Michigan State University, Lansing June 4 -15, 2007
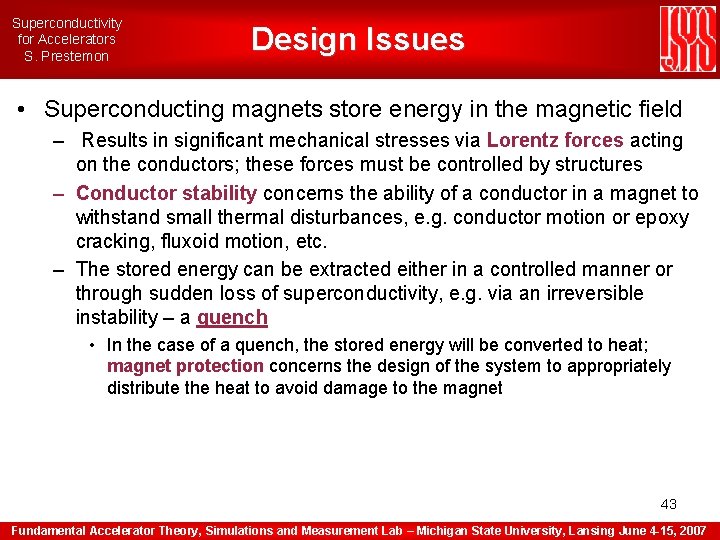
Superconductivity for Accelerators S. Prestemon Design Issues • Superconducting magnets store energy in the magnetic field – Results in significant mechanical stresses via Lorentz forces acting on the conductors; these forces must be controlled by structures – Conductor stability concerns the ability of a conductor in a magnet to withstand small thermal disturbances, e. g. conductor motion or epoxy cracking, fluxoid motion, etc. – The stored energy can be extracted either in a controlled manner or through sudden loss of superconductivity, e. g. via an irreversible instability – a quench • In the case of a quench, the stored energy will be converted to heat; magnet protection concerns the design of the system to appropriately distribute the heat to avoid damage to the magnet 43 Fundamental Accelerator Theory, Simulations and Measurement Lab – Michigan State University, Lansing June 4 -15, 2007
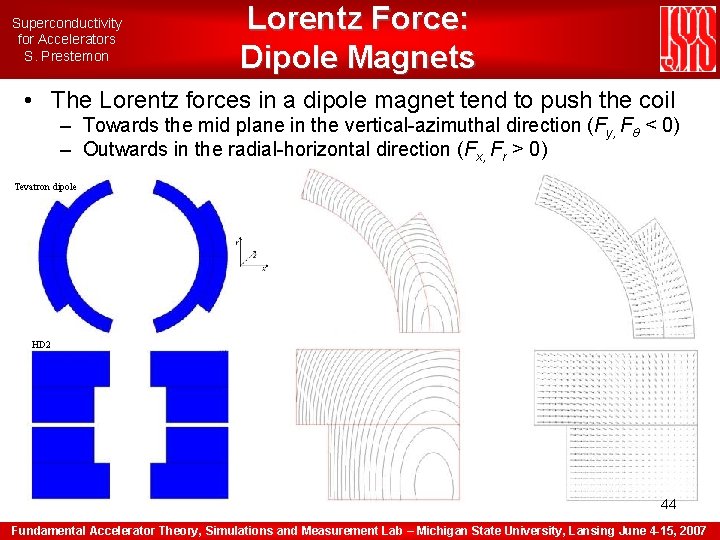
Superconductivity for Accelerators S. Prestemon Lorentz Force: Dipole Magnets • The Lorentz forces in a dipole magnet tend to push the coil – Towards the mid plane in the vertical-azimuthal direction (Fy, F < 0) – Outwards in the radial-horizontal direction (Fx, Fr > 0) Tevatron dipole HD 2 44 Fundamental Accelerator Theory, Simulations and Measurement Lab – Michigan State University, Lansing June 4 -15, 2007
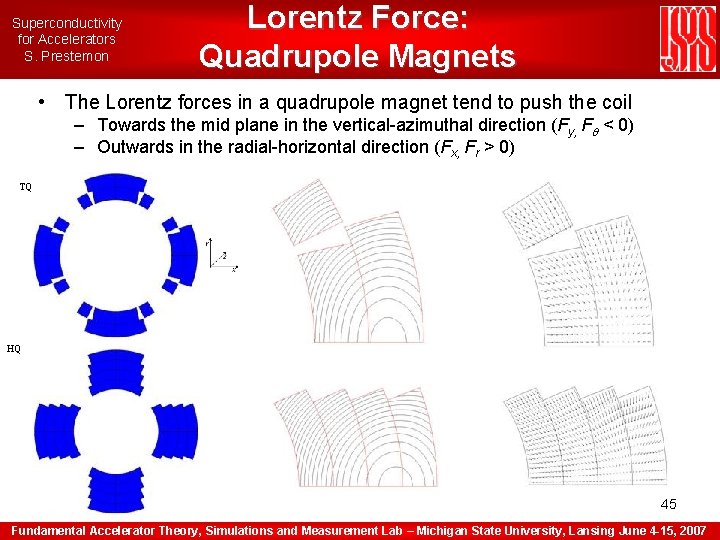
Superconductivity for Accelerators S. Prestemon Lorentz Force: Quadrupole Magnets • The Lorentz forces in a quadrupole magnet tend to push the coil – Towards the mid plane in the vertical-azimuthal direction (Fy, F < 0) – Outwards in the radial-horizontal direction (Fx, Fr > 0) TQ HQ 45 Fundamental Accelerator Theory, Simulations and Measurement Lab – Michigan State University, Lansing June 4 -15, 2007
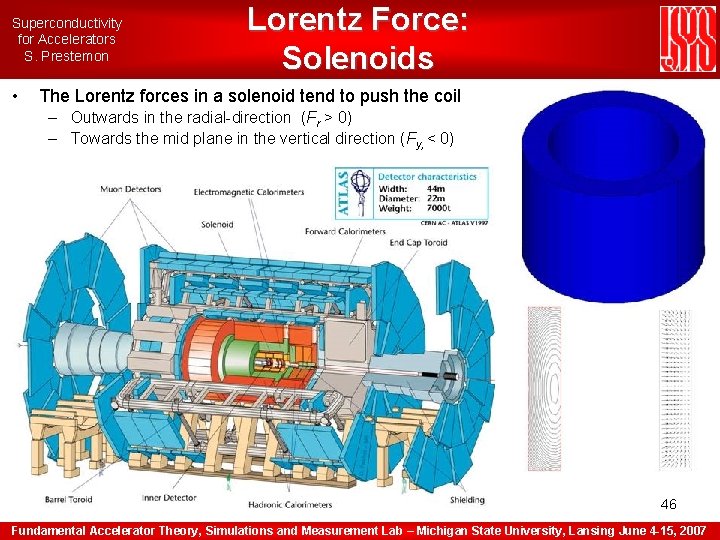
Superconductivity for Accelerators S. Prestemon • Lorentz Force: Solenoids The Lorentz forces in a solenoid tend to push the coil – Outwards in the radial-direction (Fr > 0) – Towards the mid plane in the vertical direction (Fy, < 0) 46 Fundamental Accelerator Theory, Simulations and Measurement Lab – Michigan State University, Lansing June 4 -15, 2007
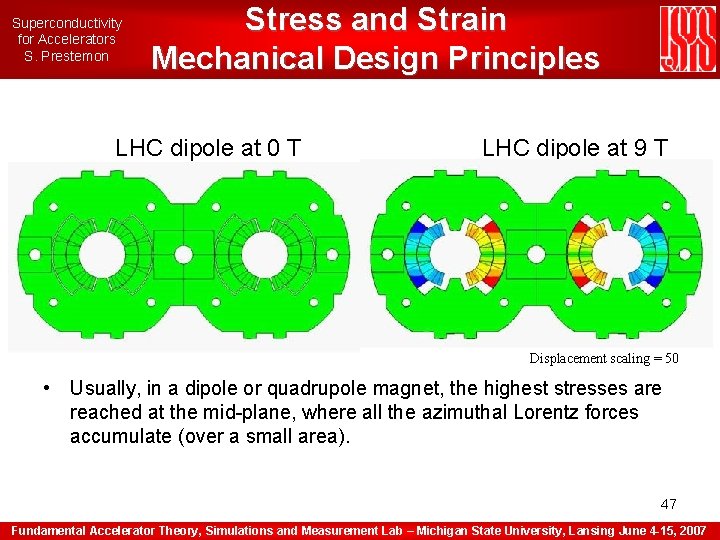
Superconductivity for Accelerators S. Prestemon Stress and Strain Mechanical Design Principles LHC dipole at 0 T LHC dipole at 9 T Displacement scaling = 50 • Usually, in a dipole or quadrupole magnet, the highest stresses are reached at the mid-plane, where all the azimuthal Lorentz forces accumulate (over a small area). 47 Fundamental Accelerator Theory, Simulations and Measurement Lab – Michigan State University, Lansing June 4 -15, 2007
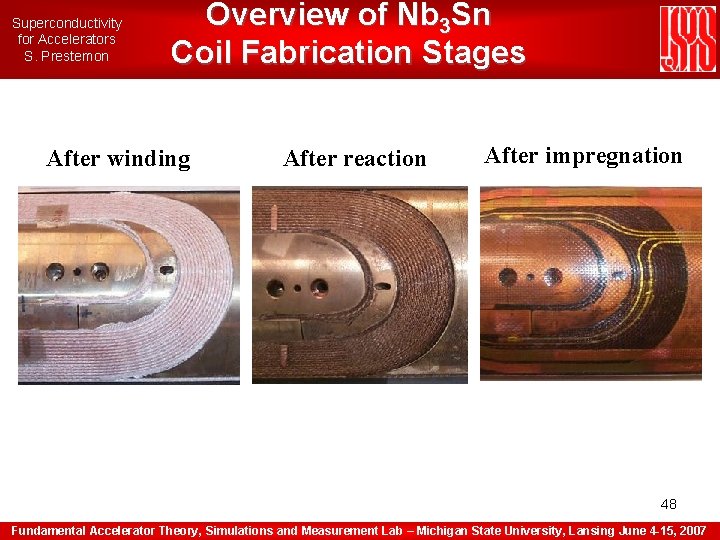
Superconductivity for Accelerators S. Prestemon Overview of Nb 3 Sn Coil Fabrication Stages After winding After reaction Cured with matrix Reacted After impregnation Epoxy impregnated 48 Fundamental Accelerator Theory, Simulations and Measurement Lab – Michigan State University, Lansing June 4 -15, 2007
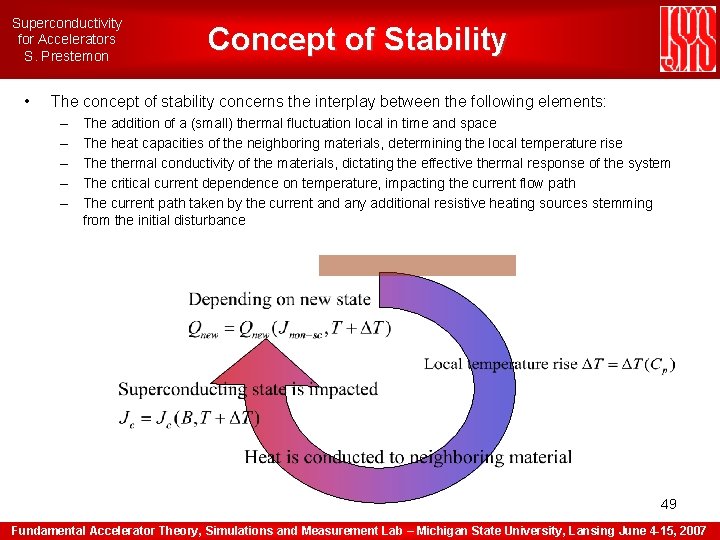
Superconductivity for Accelerators S. Prestemon • Concept of Stability The concept of stability concerns the interplay between the following elements: – – – The addition of a (small) thermal fluctuation local in time and space The heat capacities of the neighboring materials, determining the local temperature rise The thermal conductivity of the materials, dictating the effective thermal response of the system The critical current dependence on temperature, impacting the current flow path The current path taken by the current and any additional resistive heating sources stemming from the initial disturbance 49 Fundamental Accelerator Theory, Simulations and Measurement Lab – Michigan State University, Lansing June 4 -15, 2007
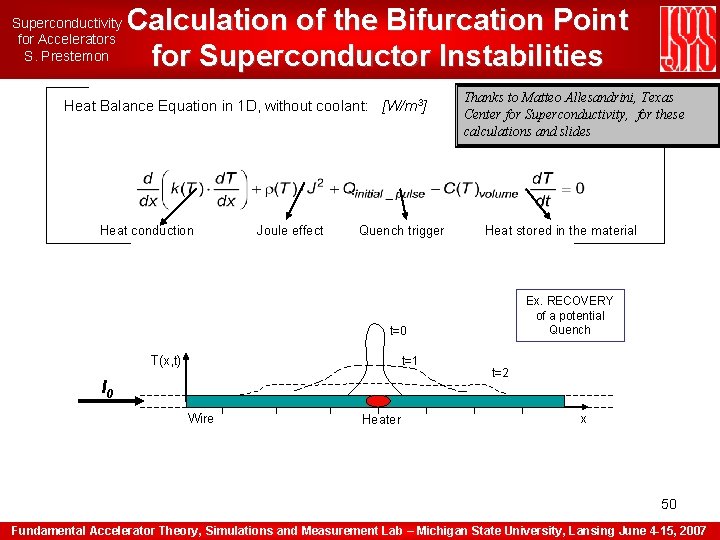
Superconductivity for Accelerators S. Prestemon Calculation of the Bifurcation Point for Superconductor Instabilities Heat Balance Equation in 1 D, without coolant: [W/m 3] Heat conduction Joule effect Quench trigger Thanks to Matteo Allesandrini, Texas Center for Superconductivity, for these calculations and slides Heat stored in the material Ex. RECOVERY of a potential Quench t=0 T(x, t) t=1 I 0 Wire Heater t=2 x 50 Fundamental Accelerator Theory, Simulations and Measurement Lab – Michigan State University, Lansing June 4 -15, 2007
![Superconductivity for Accelerators S Prestemon Analysis of SQ 02 QUENCH with 1 m J Superconductivity for Accelerators S. Prestemon Analysis of SQ 02 QUENCH with 1 [m. J]](https://slidetodoc.com/presentation_image_h2/31365df123dbc3156dad0acbdf941176/image-51.jpg)
Superconductivity for Accelerators S. Prestemon Analysis of SQ 02 QUENCH with 1 [m. J] Linear Scale Quench Temperature [K] at ion e H sit po e d Len gth [m] Time [s] 51 Fundamental Accelerator Theory, Simulations and Measurement Lab – Michigan State University, Lansing June 4 -15, 2007
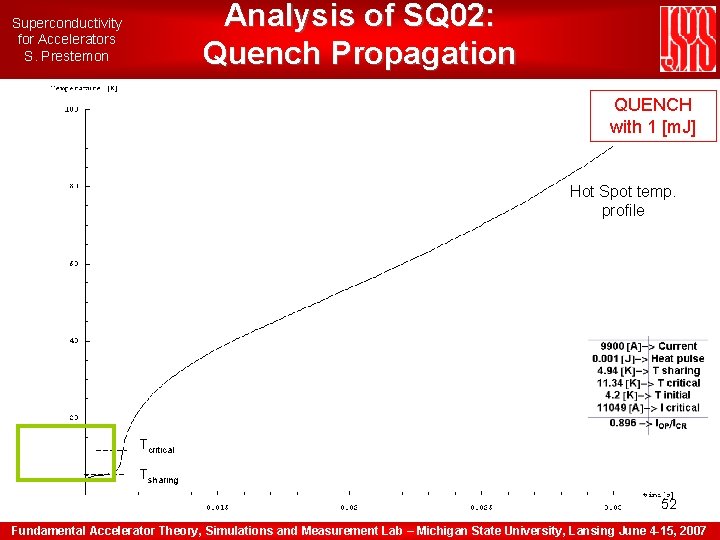
Analysis of SQ 02: Quench Propagation Superconductivity for Accelerators S. Prestemon QUENCH with 1 [m. J] Hot Spot temp. profile Tcritical Tsharing 52 Fundamental Accelerator Theory, Simulations and Measurement Lab – Michigan State University, Lansing June 4 -15, 2007
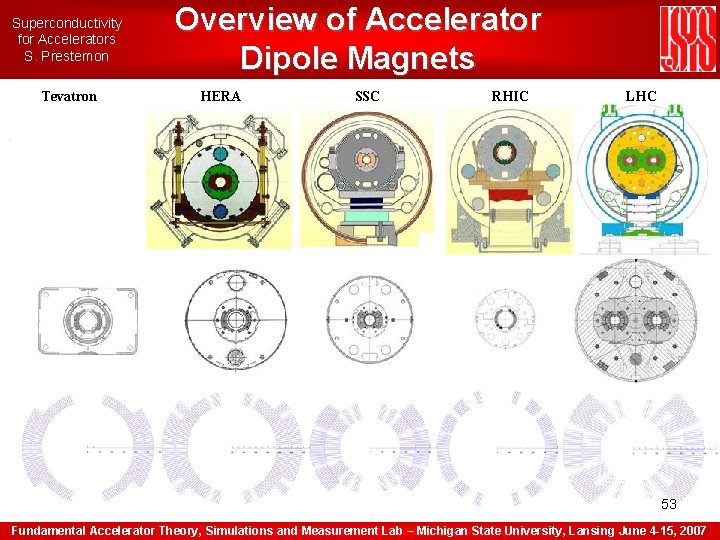
Superconductivity for Accelerators S. Prestemon Tevatron Overview of Accelerator Dipole Magnets HERA SSC RHIC LHC 53 Fundamental Accelerator Theory, Simulations and Measurement Lab – Michigan State University, Lansing June 4 -15, 2007
Soren prestemon
Soren prestemon
Soren prestemon
Kotter 8 accelerators
Set current query acceleration
Good to great technology accelerators
The long-term future of particle accelerators
Good to great chapter 7
Accelerators computer architecture
Cosmic super accelerators
Superconductivity
Superconductivity
Superconductivity introduction
Superconductivity
Superconductivity
Hubbard
Soren kaplan
Superconductivity a level physics
Morenzoni
Superconductivity
Karen soren
Superconductivity
Superconductivity
Superconductivity
Soren comp
01:640:244 lecture notes - lecture 15: plat, idah, farad
Mat för idrottare
Teckenspråk minoritetsspråk argument
Myndigheten för delaktighet
Svenskt ramverk för digital samverkan
Tack för att ni lyssnade
Datorkunskap för nybörjare
Kontinuitetshantering
Tobinskatten för och nackdelar
Ministerstyre för och nackdelar
Atmosfr
Mitos steg
Claes martinsson
Nyckelkompetenser för livslångt lärande
Rbk fuktmätning
Tidbok yrkesförare
Elektronik för barn
Kung dog 1611
Tack för att ni har lyssnat
Verktyg för automatisering av utbetalningar
Vem räknas som jude
Frgar
Karttecken brun triangel
Jag har gått inunder stjärnor text
Multiplikation uppställning
Stig kerman
Blomman för dagen drog
Typiska drag för en novell
Shingelfrisyren