FRCR Physics Lectures The Physics of Diagnostic Ultrasound
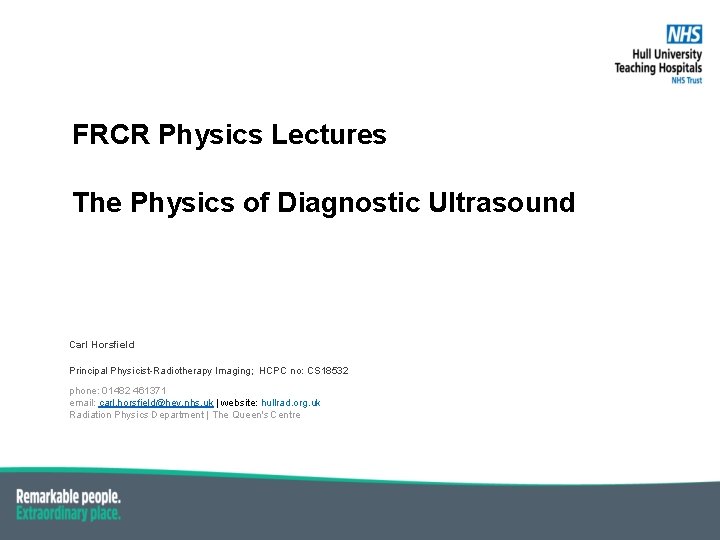
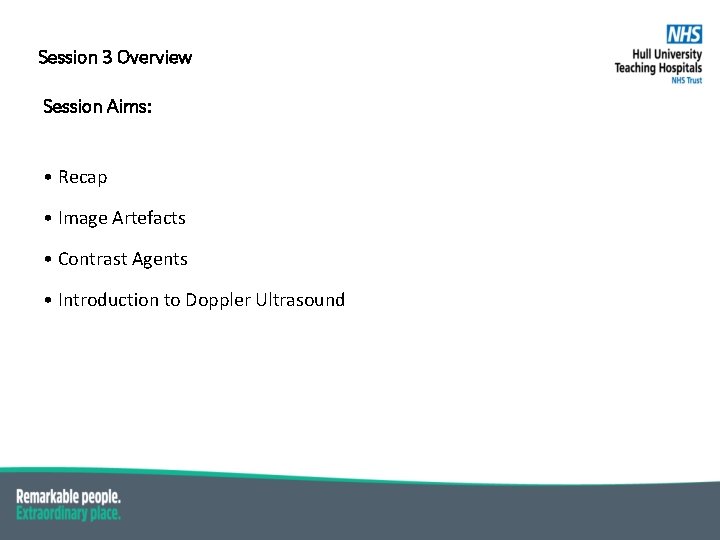
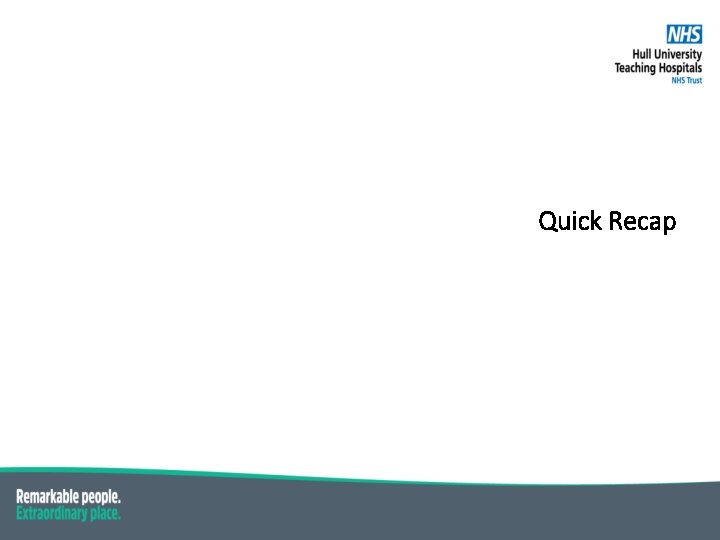
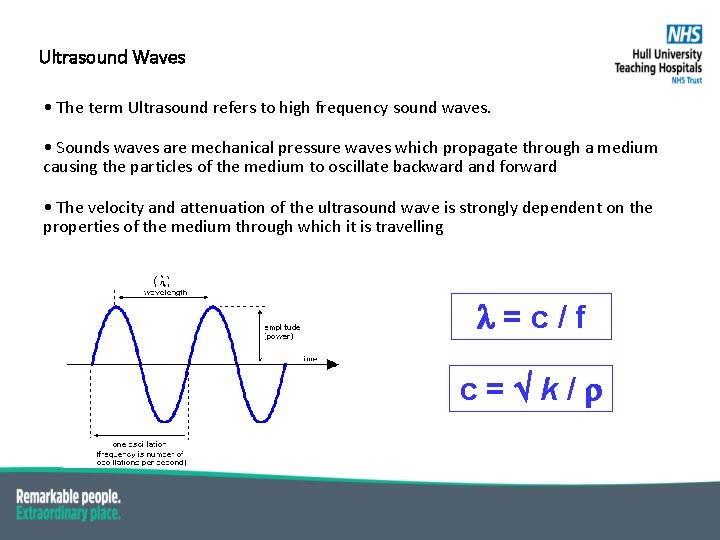
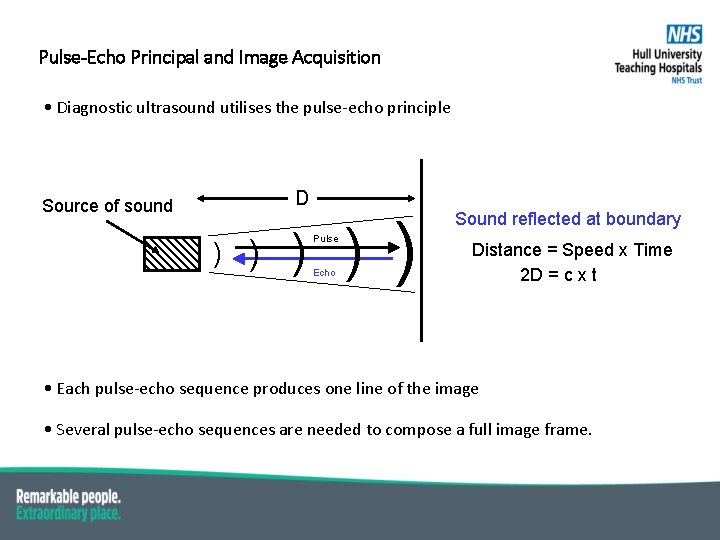
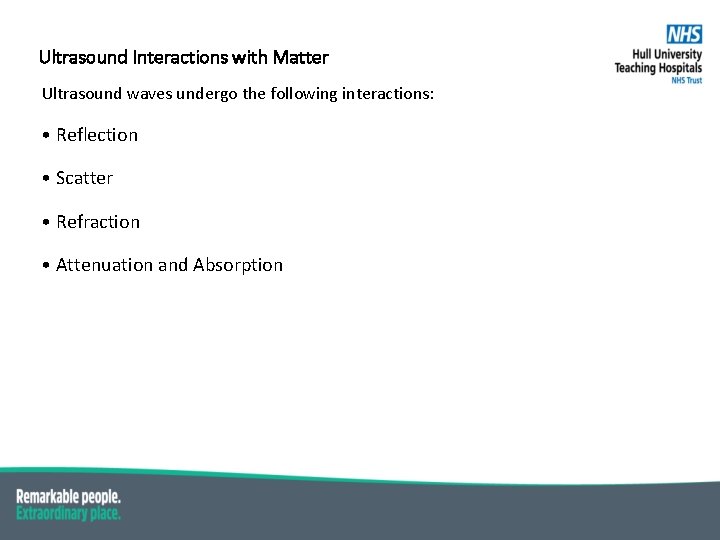
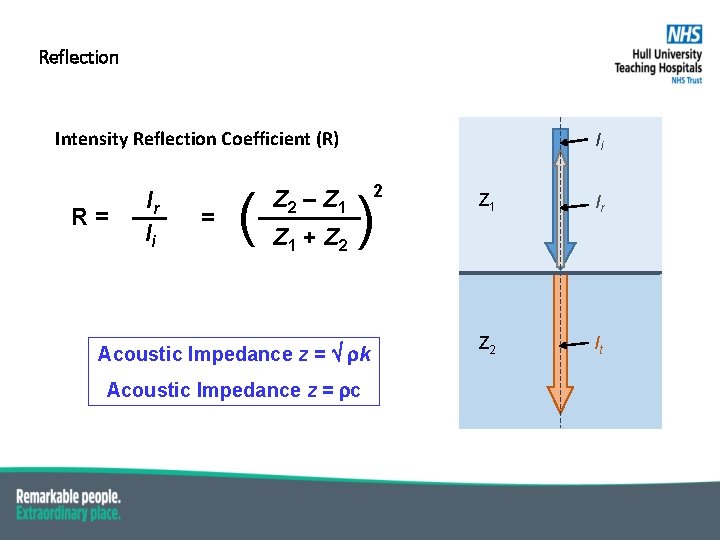
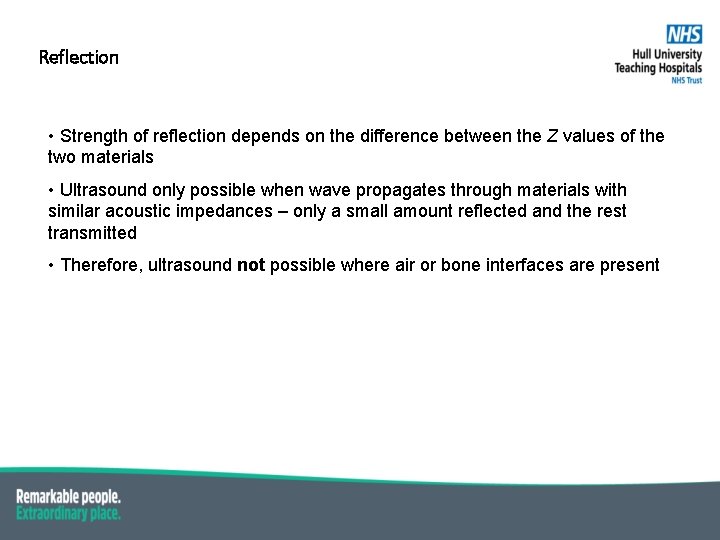
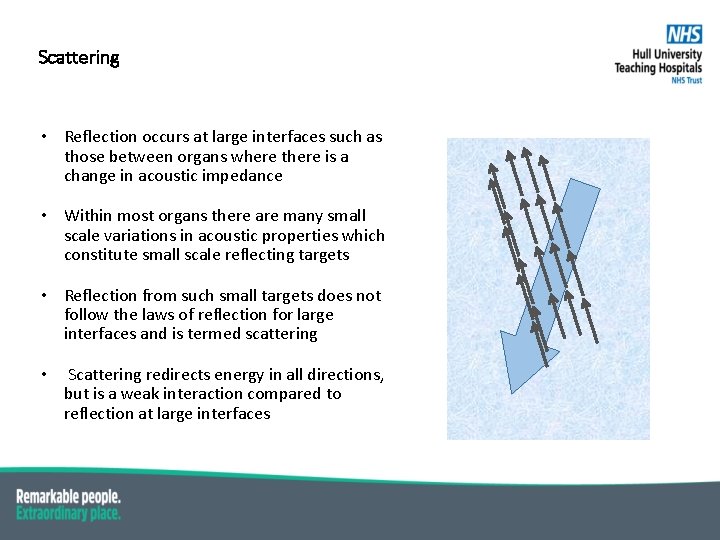
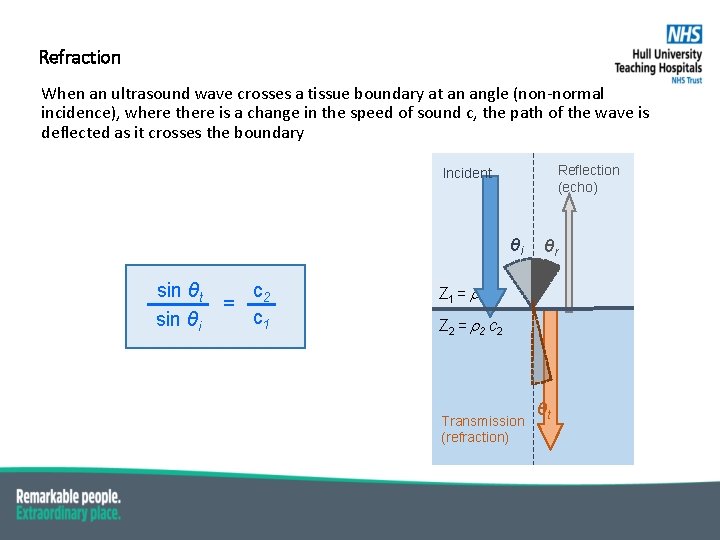
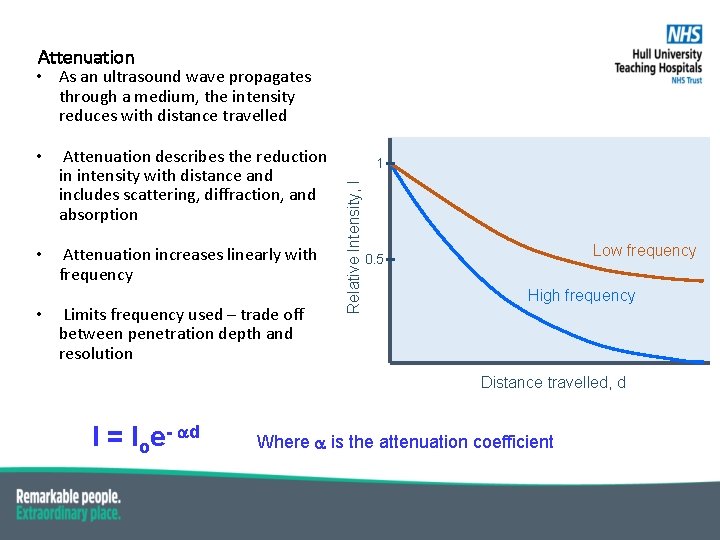
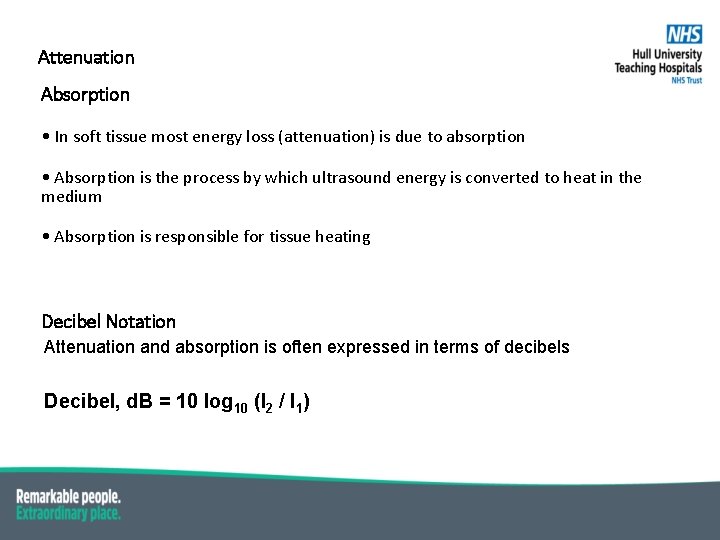
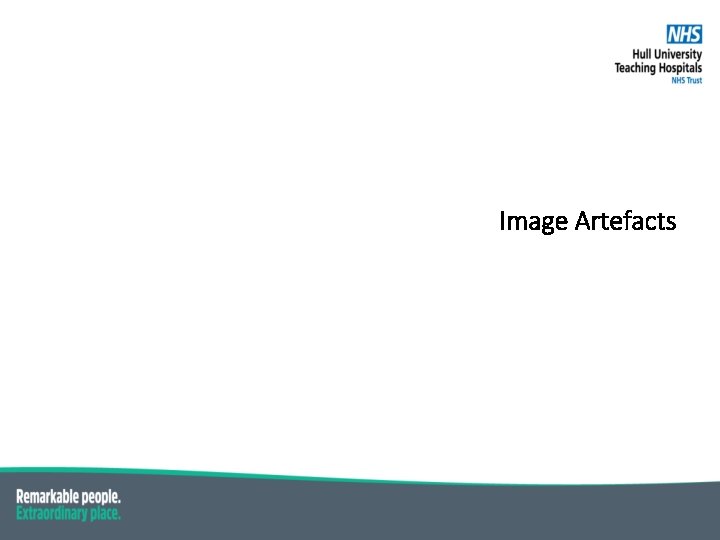
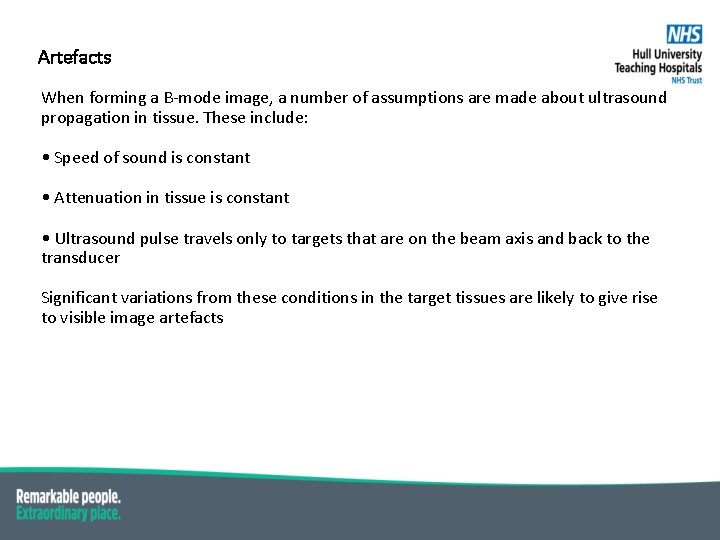
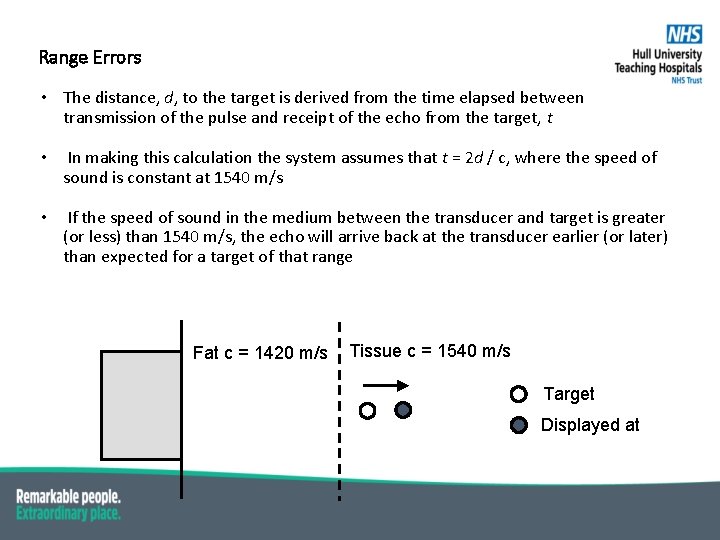
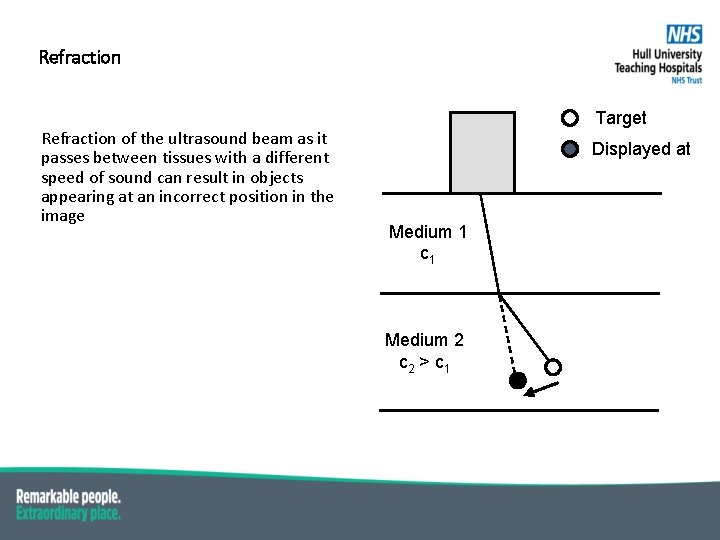
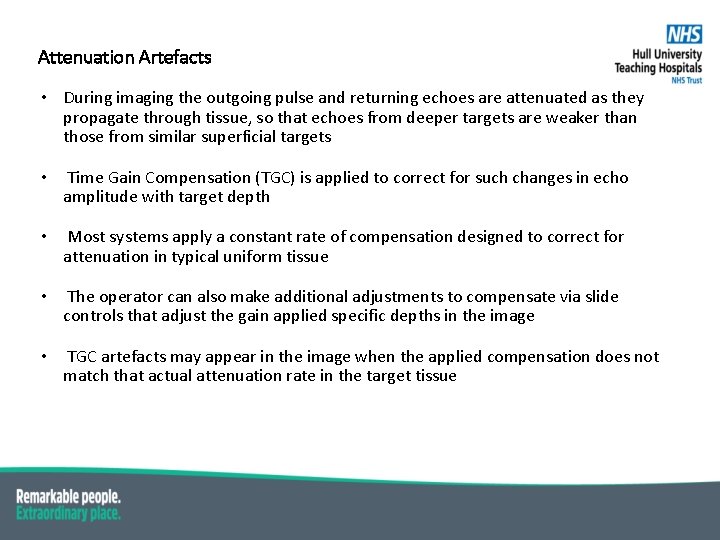
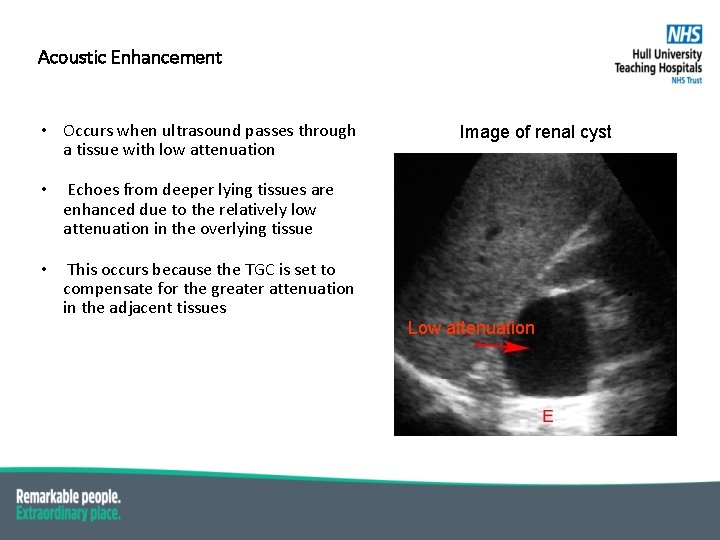
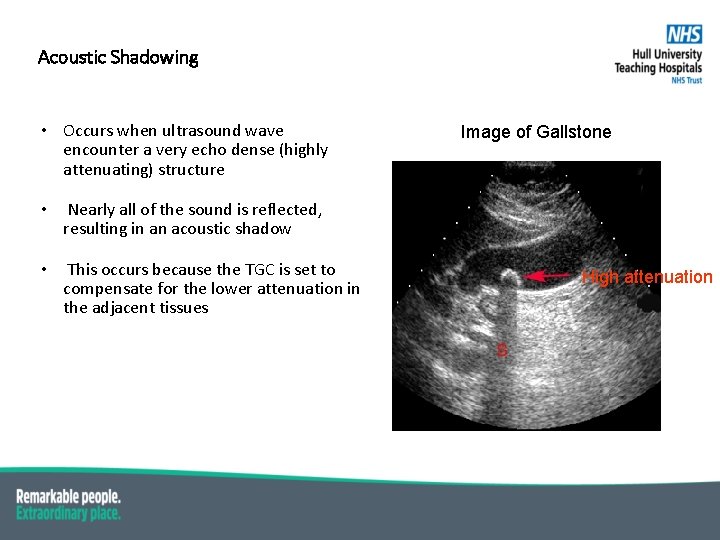
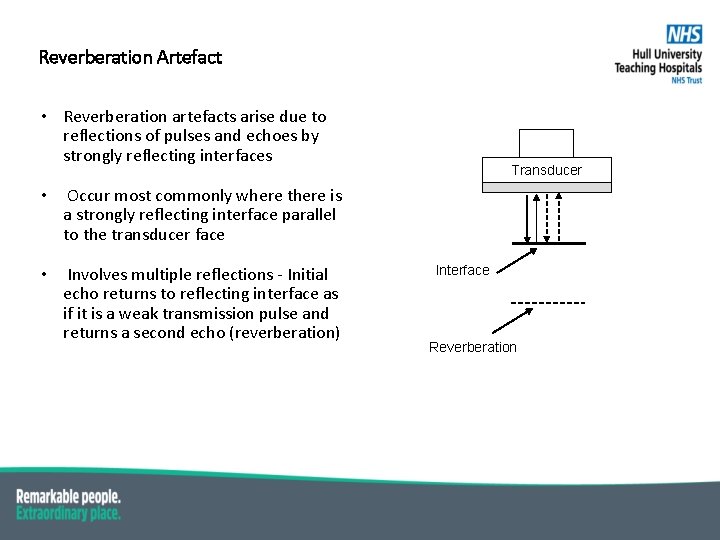
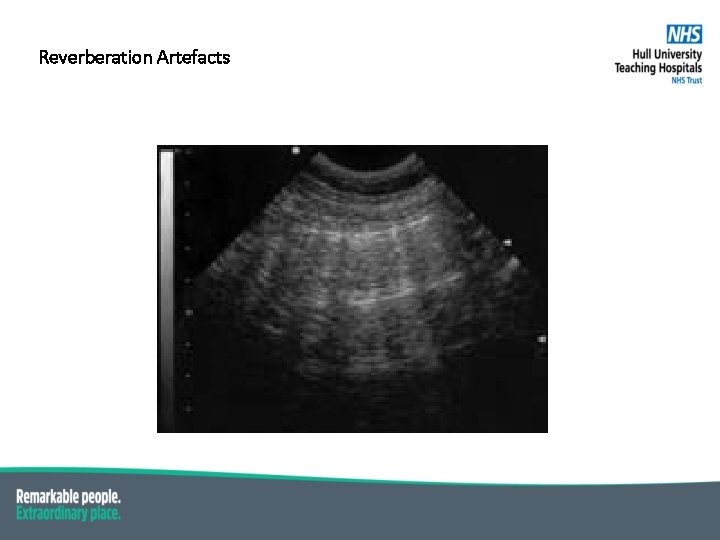
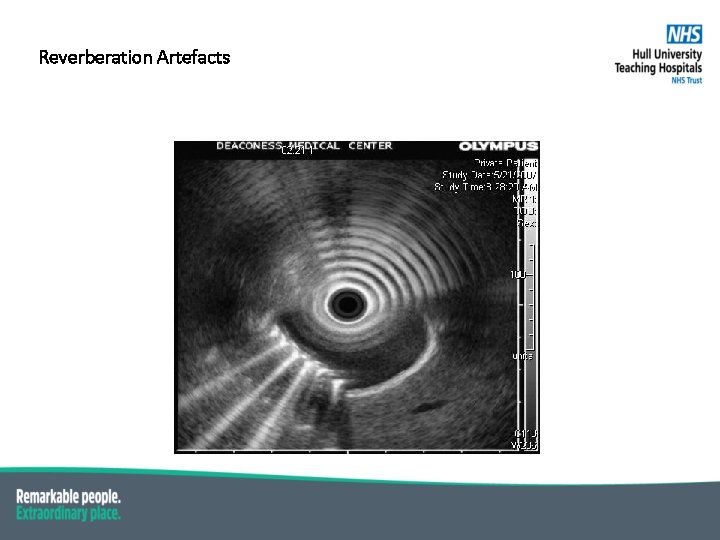
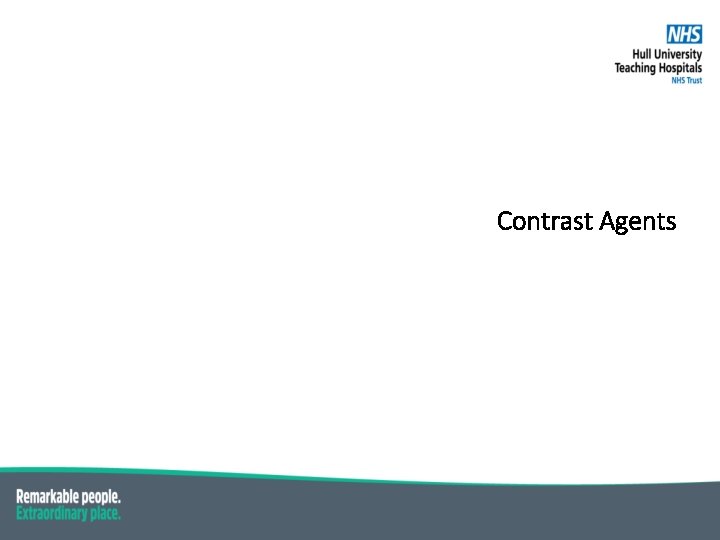
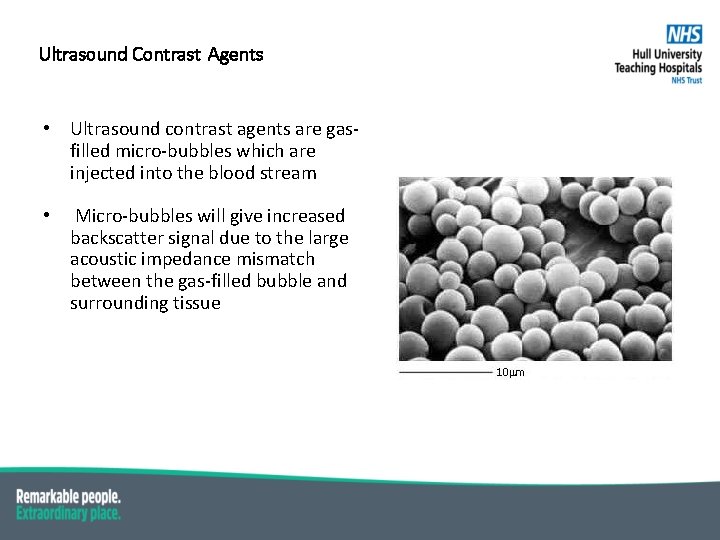
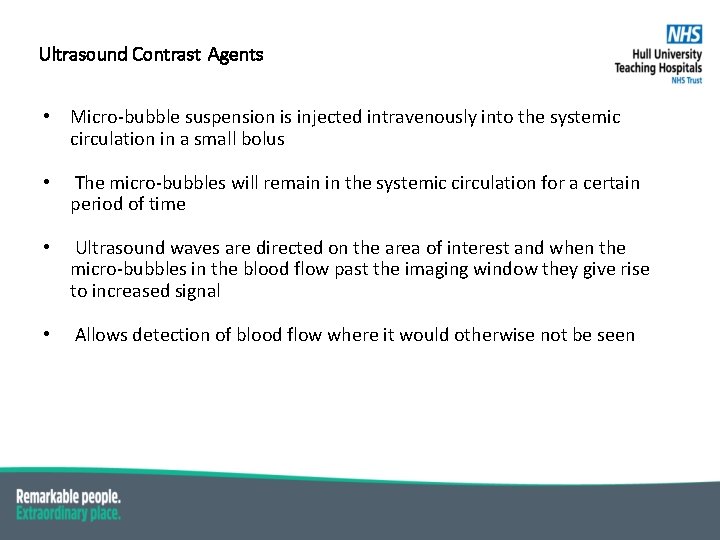
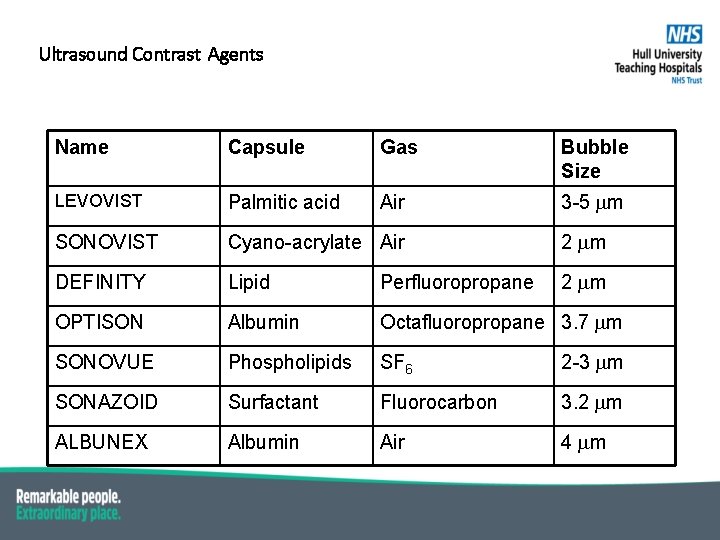
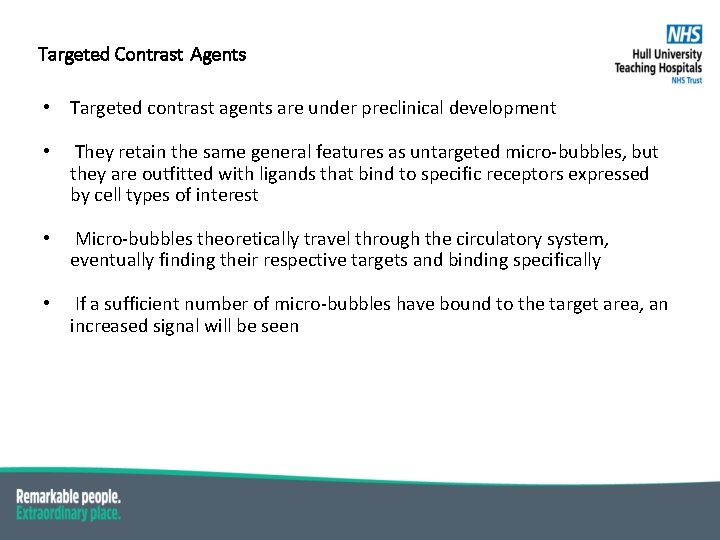
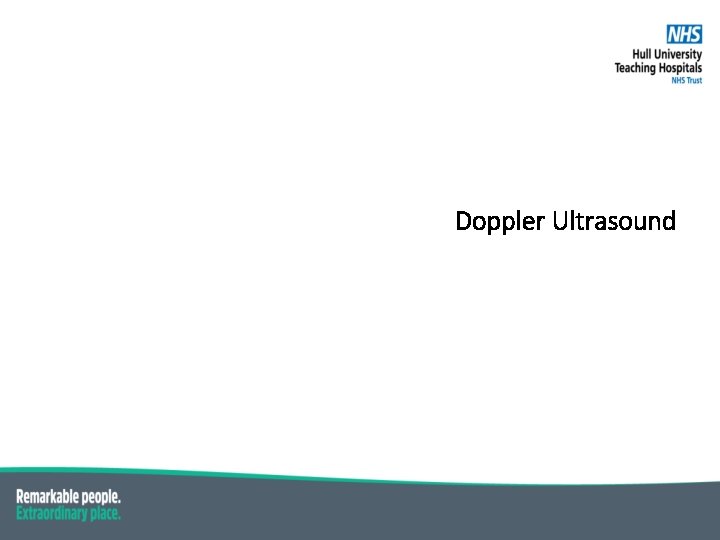
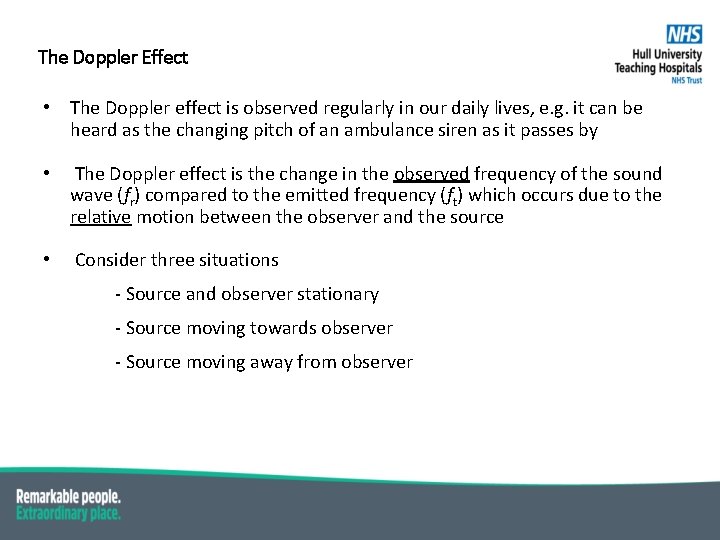
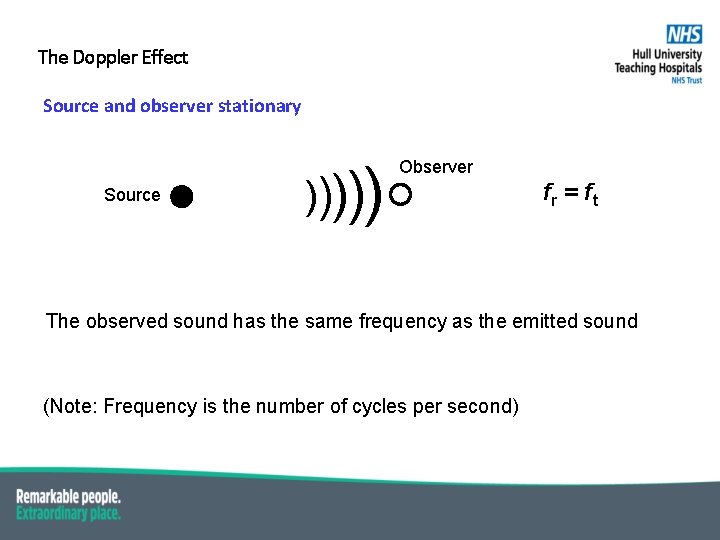
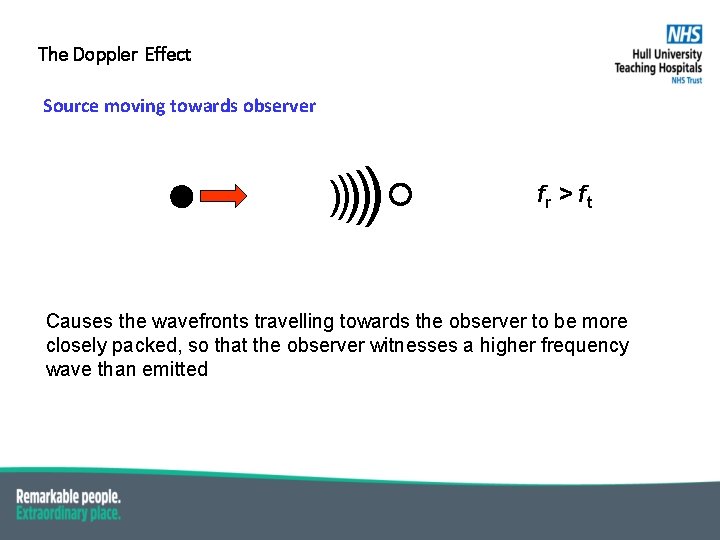
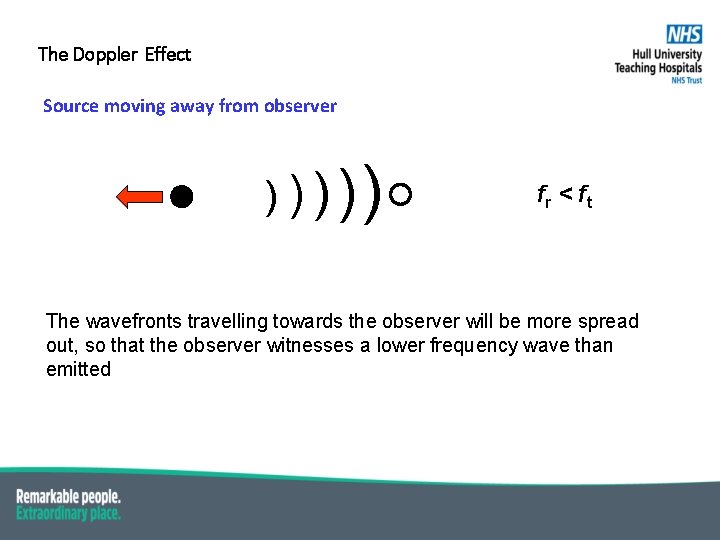
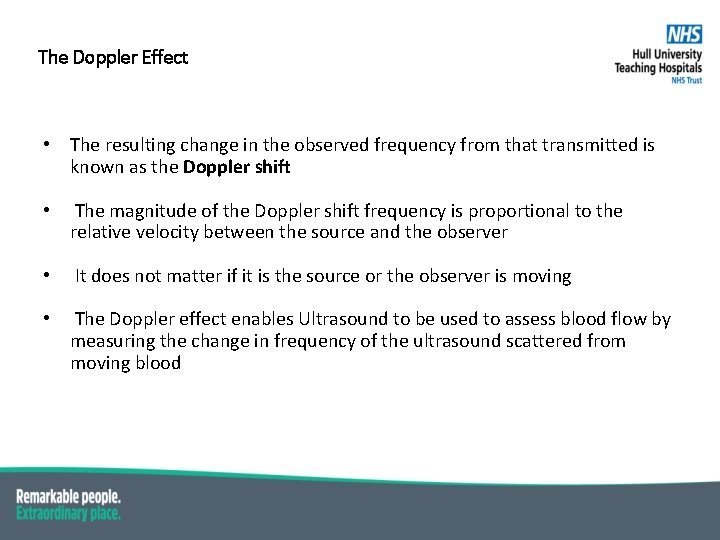
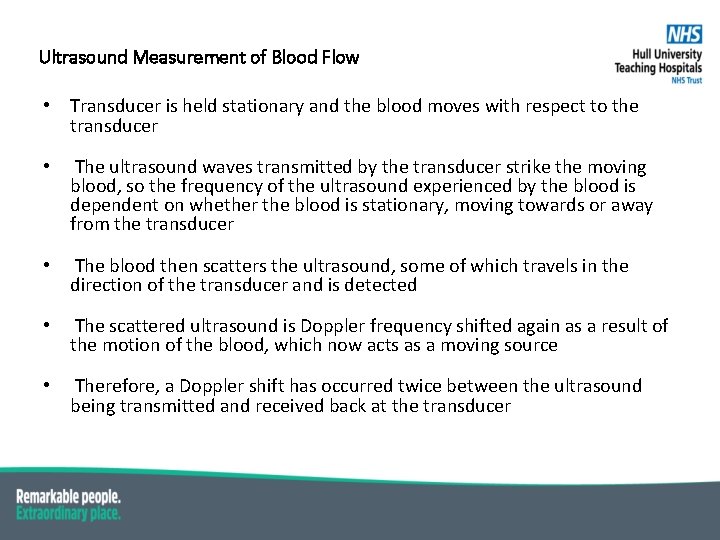
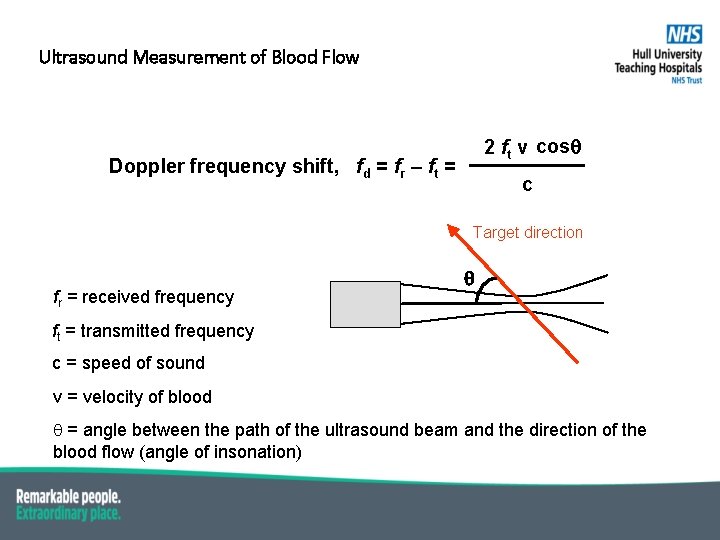
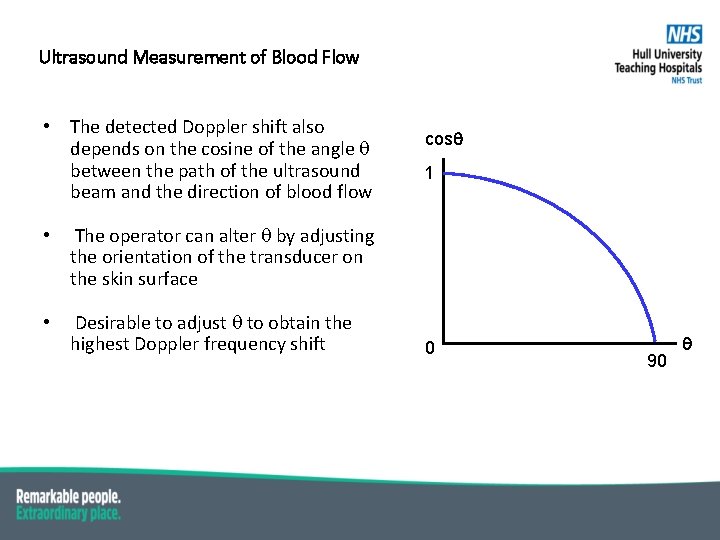
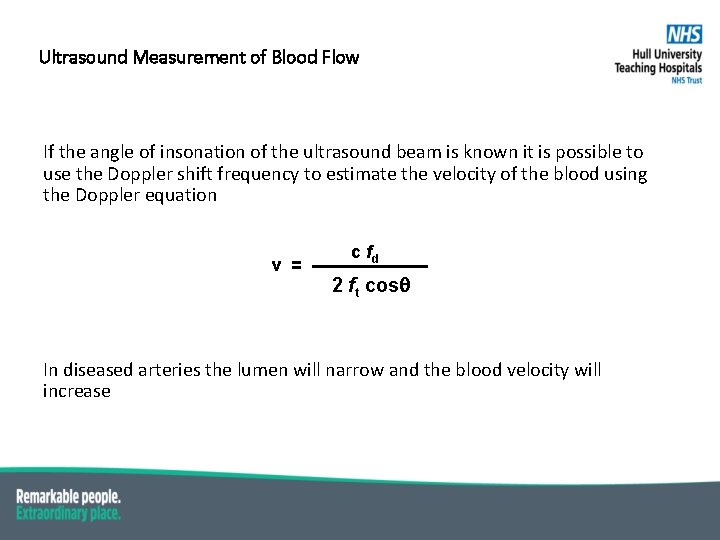
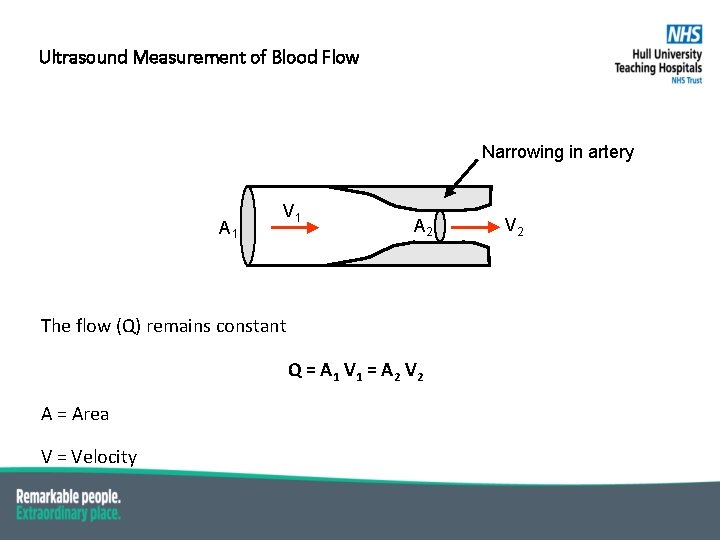
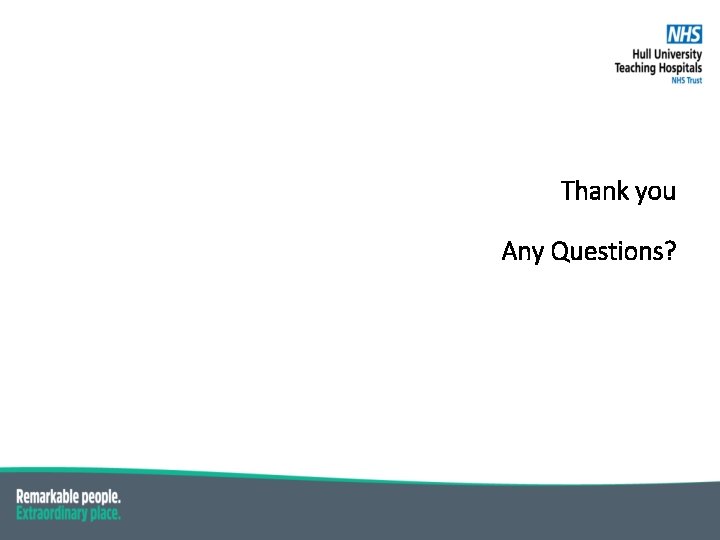
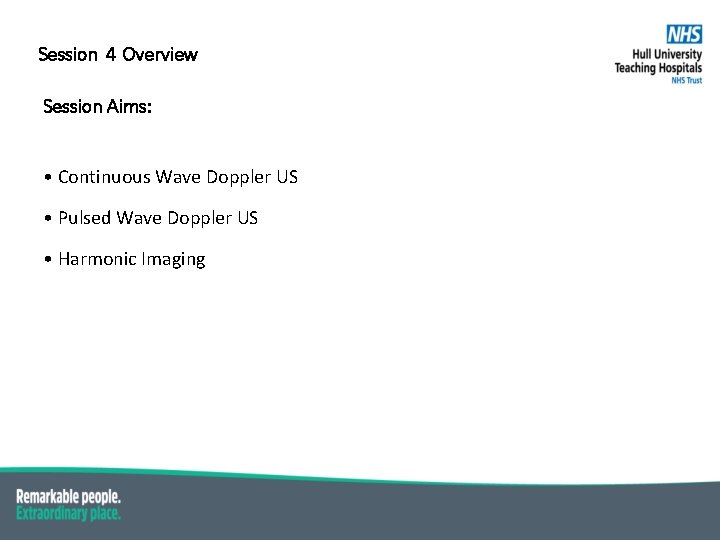
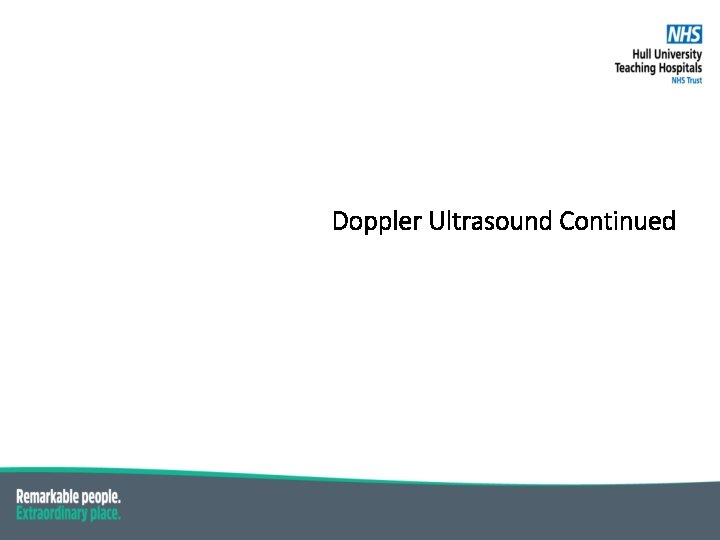
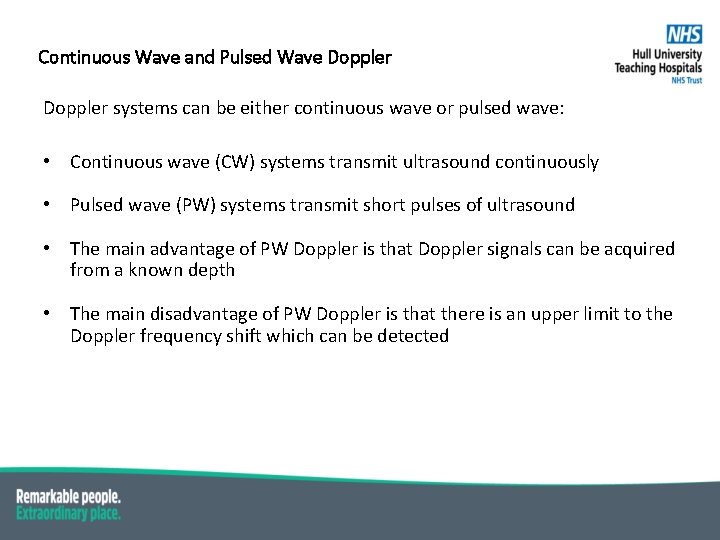
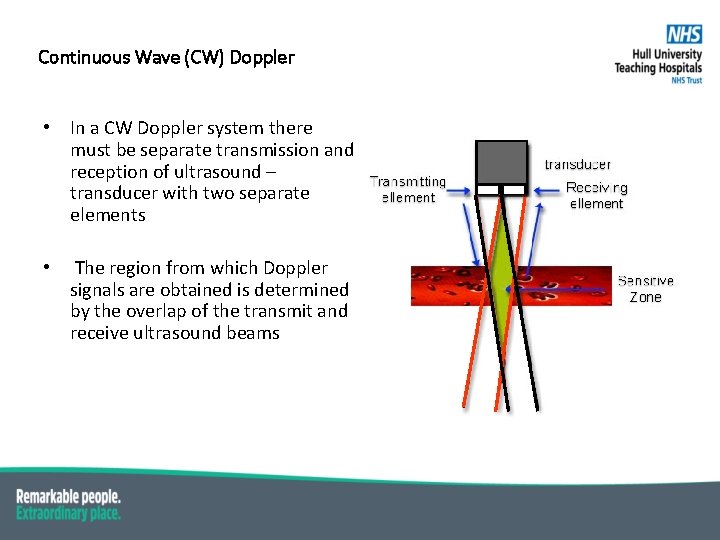
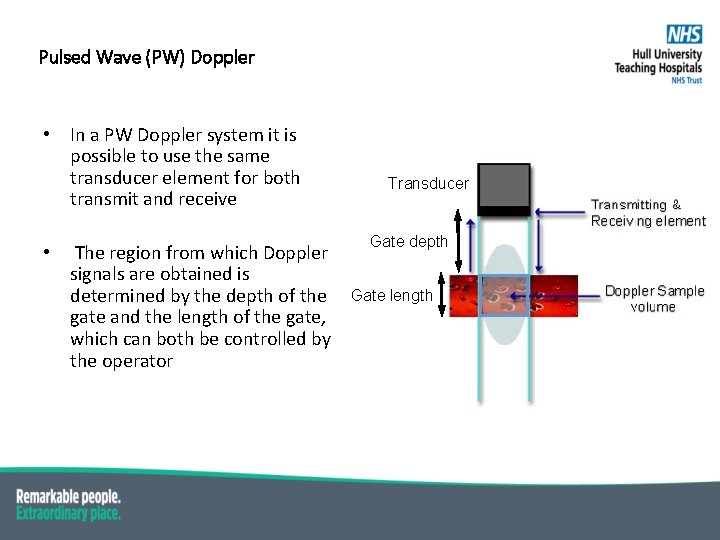
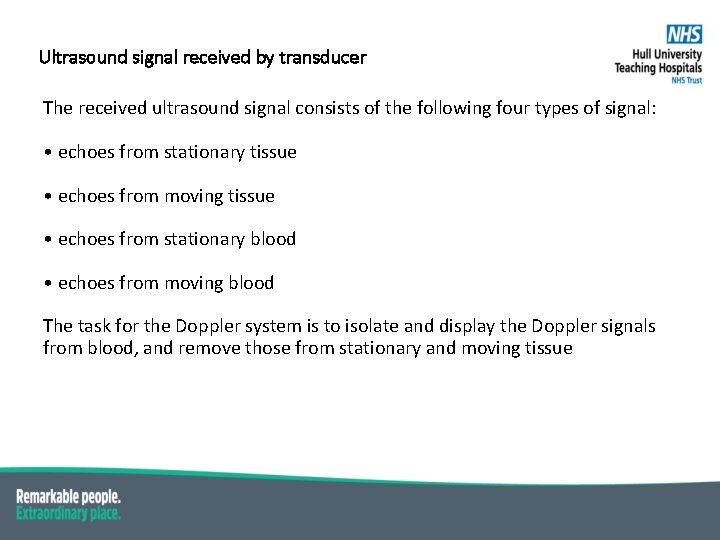
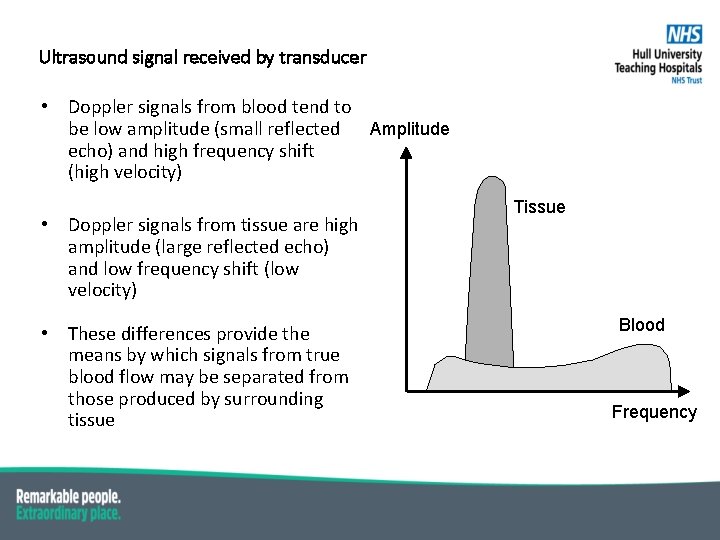
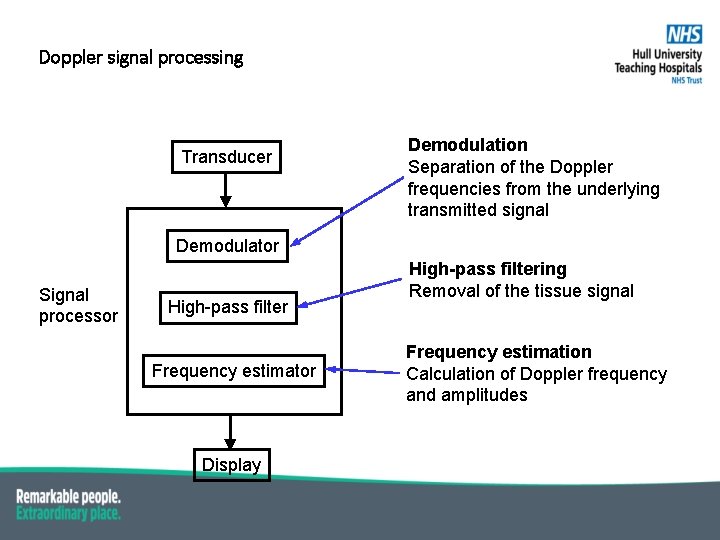
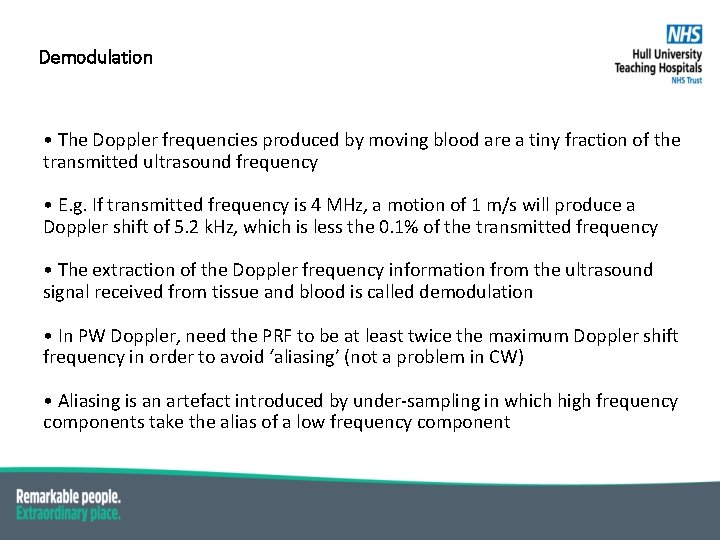
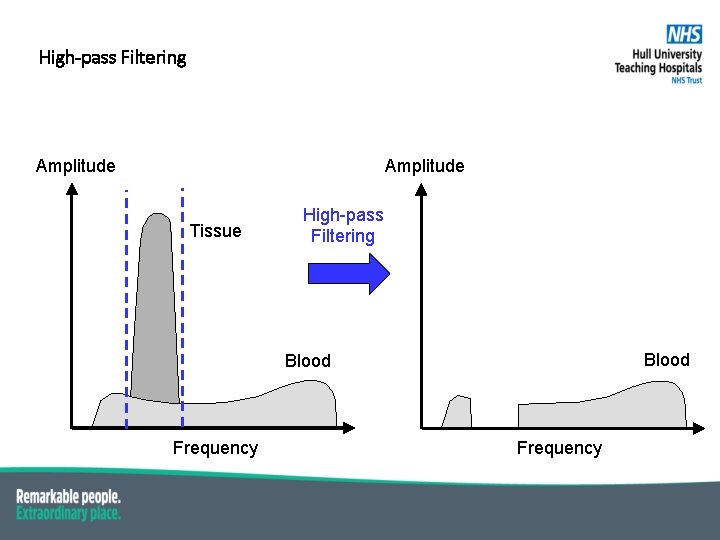
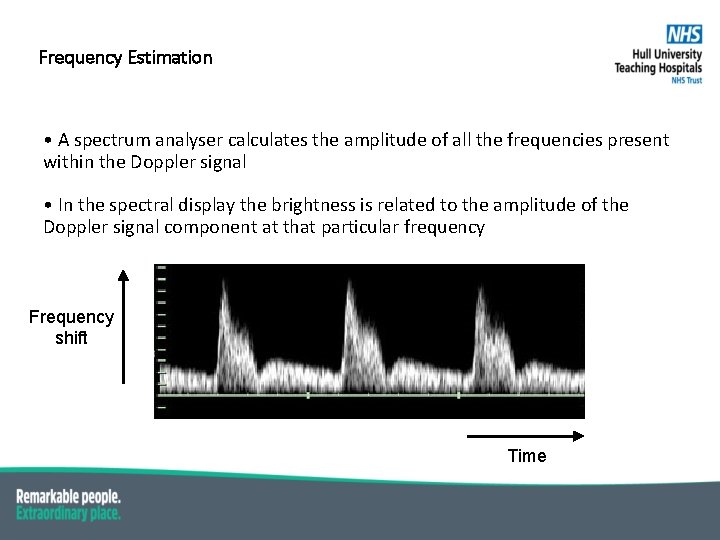
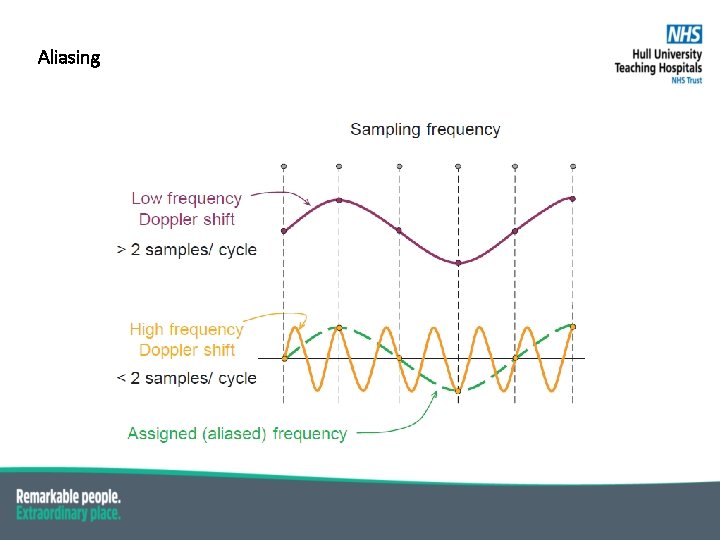
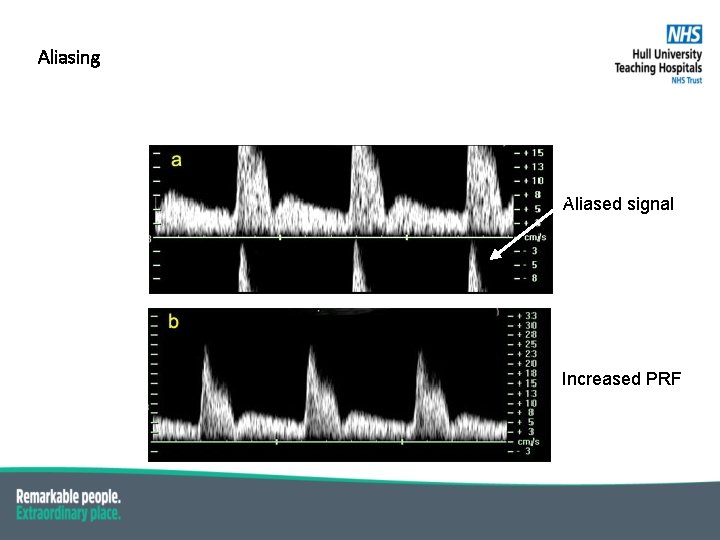
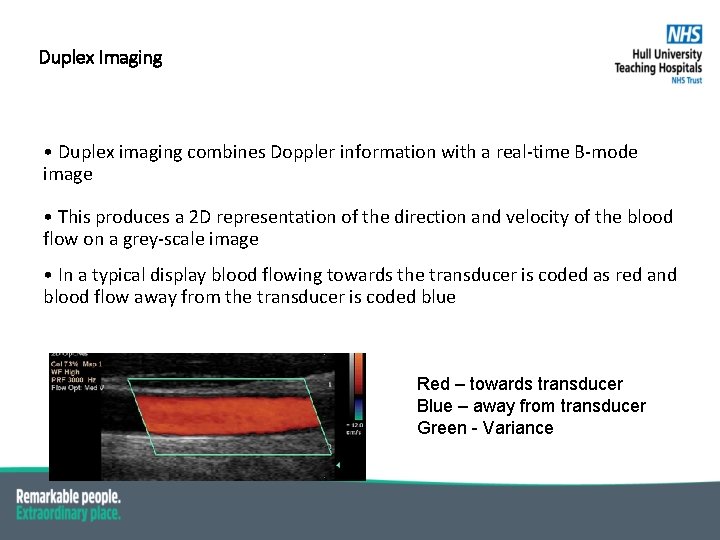
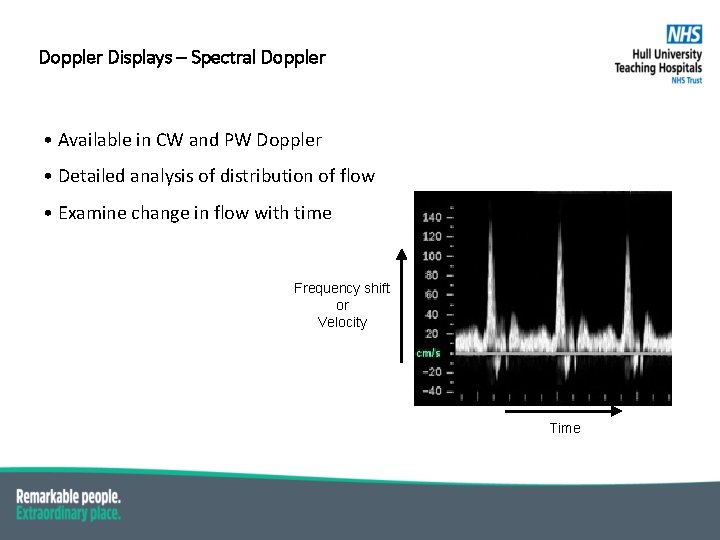
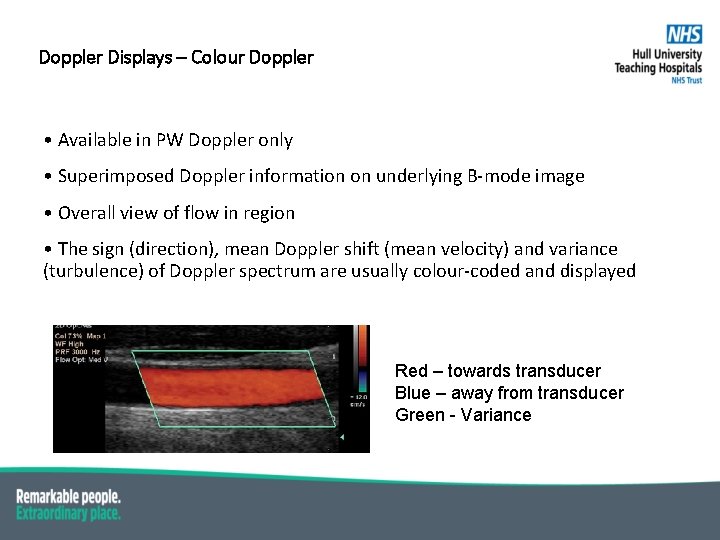
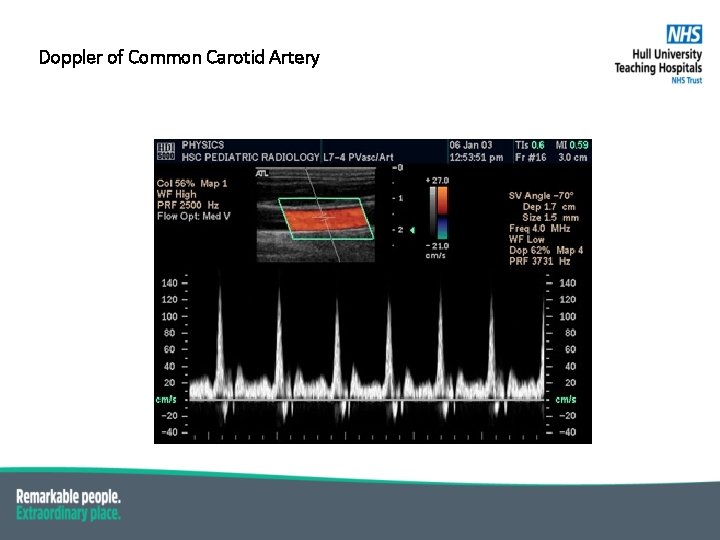
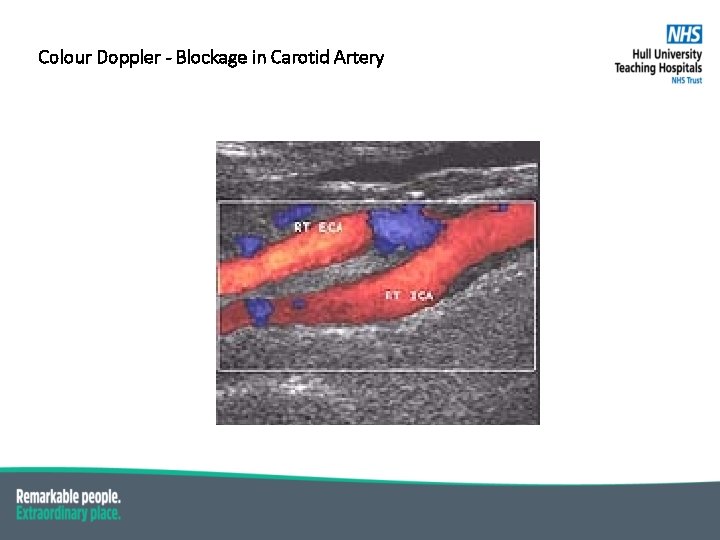
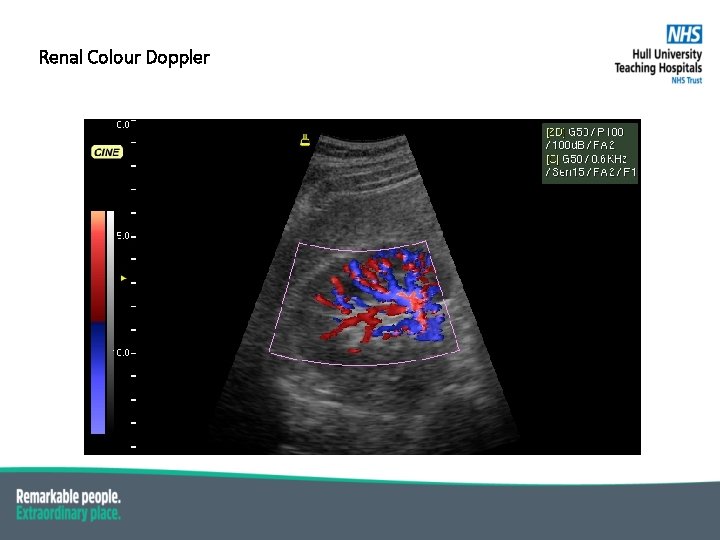
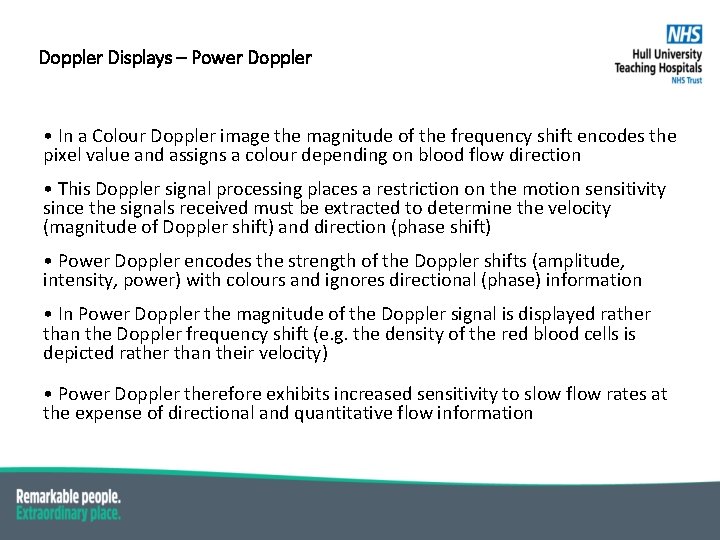
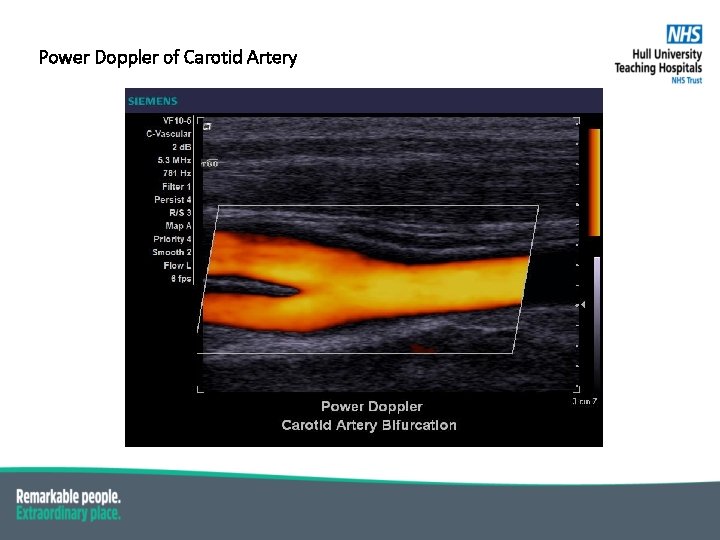
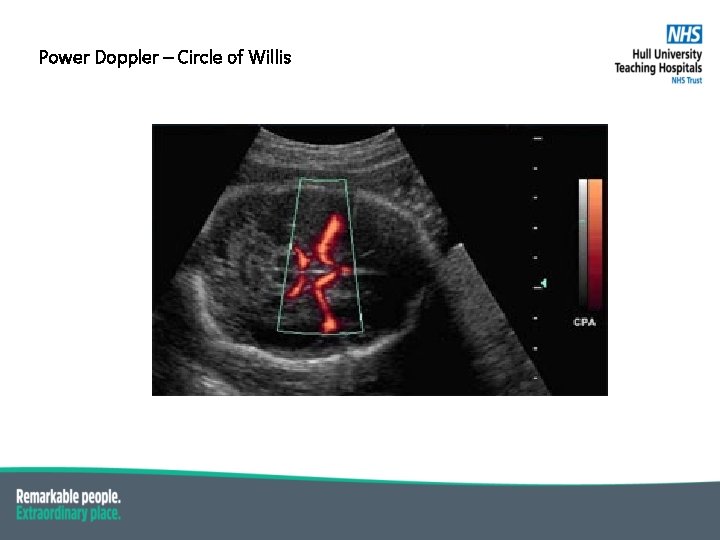
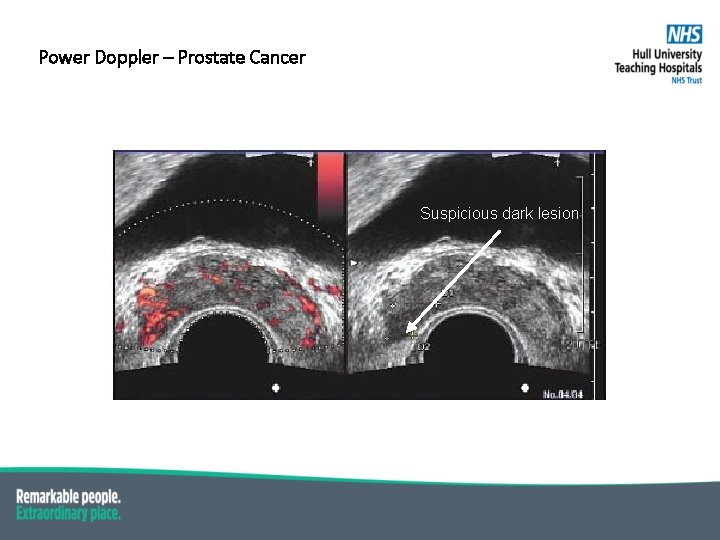
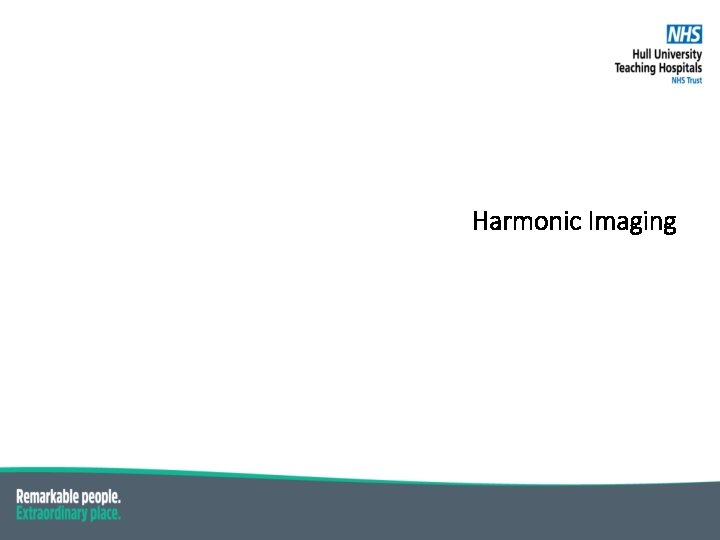
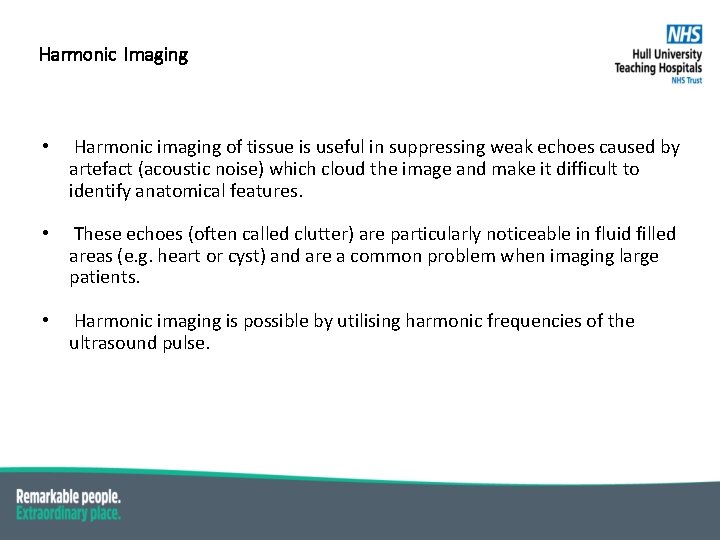
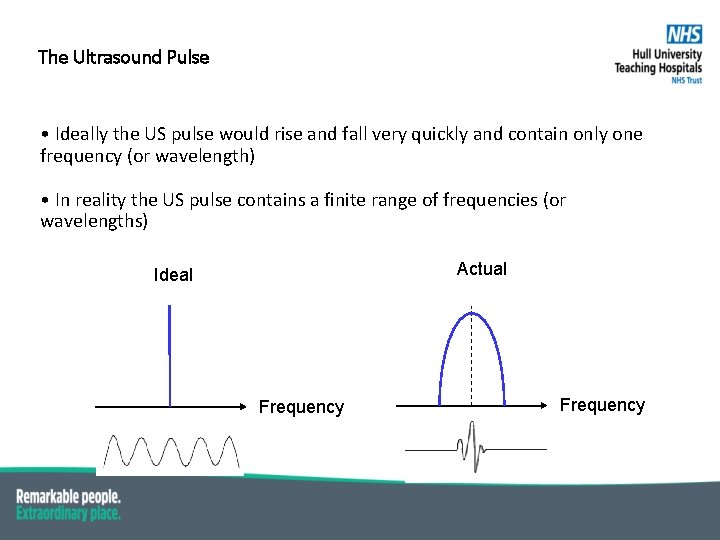
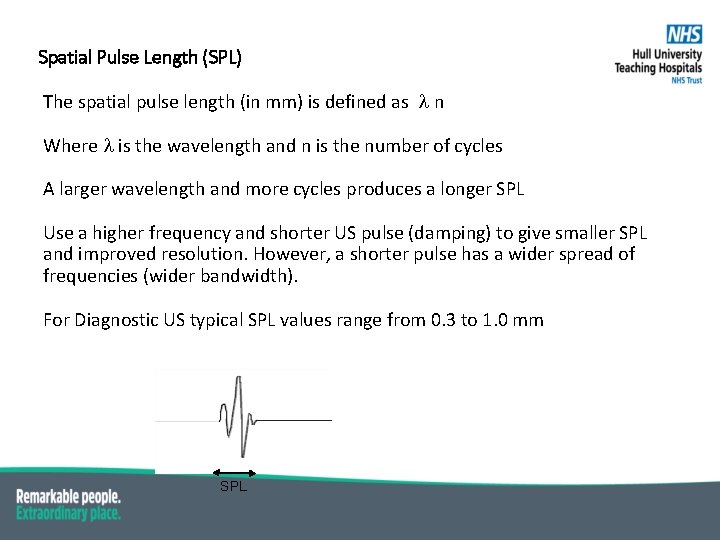
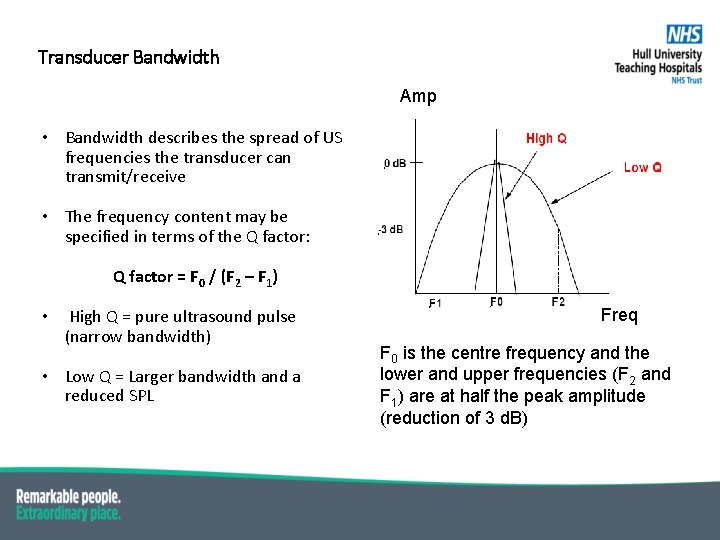
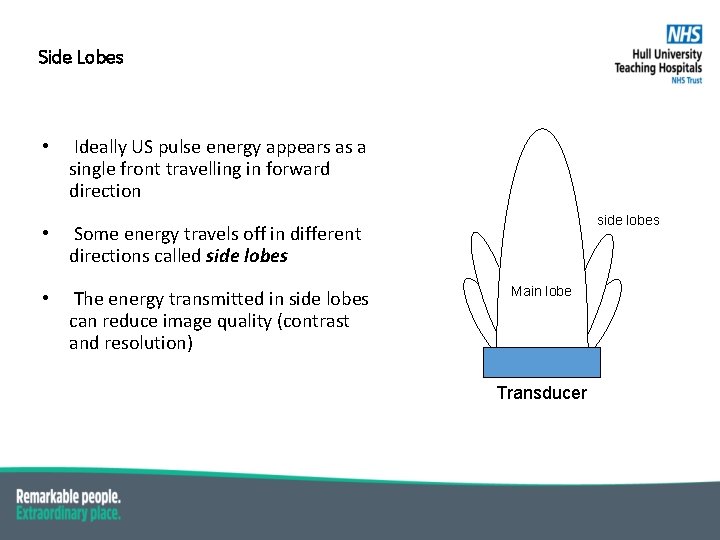
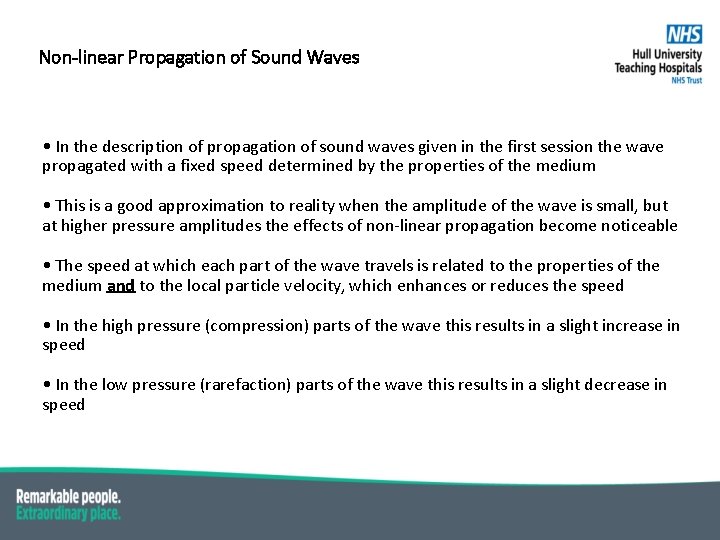
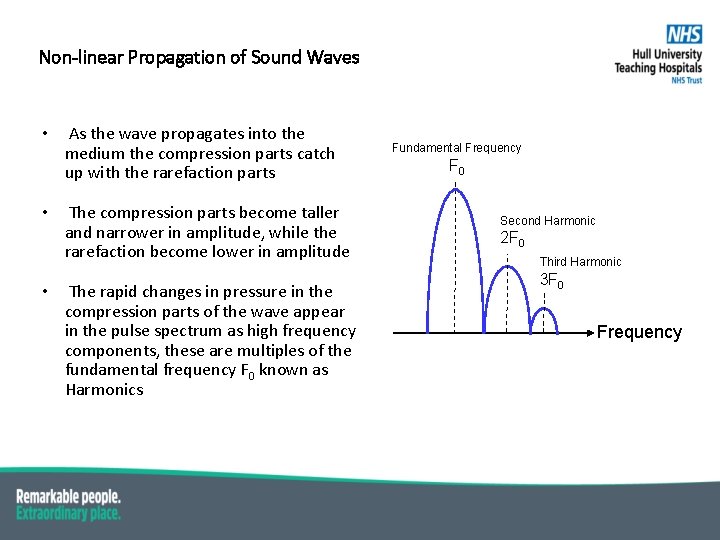
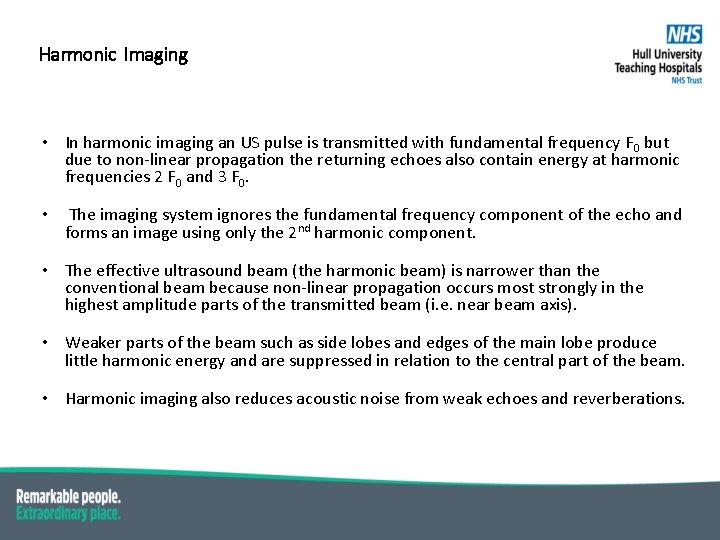
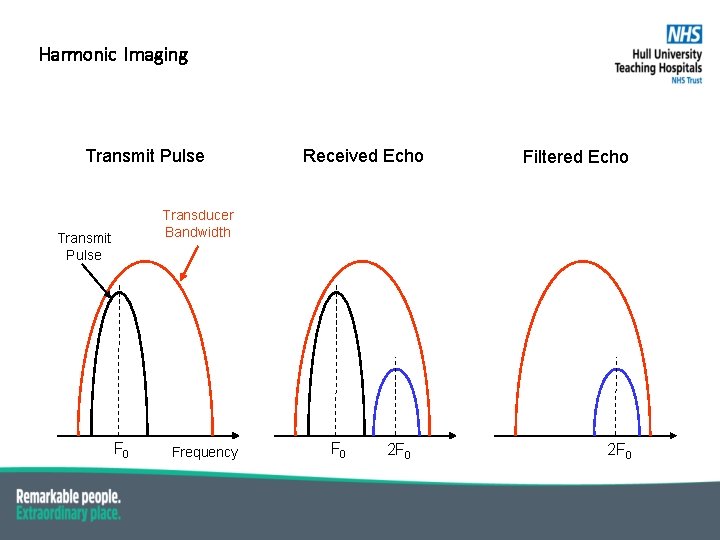
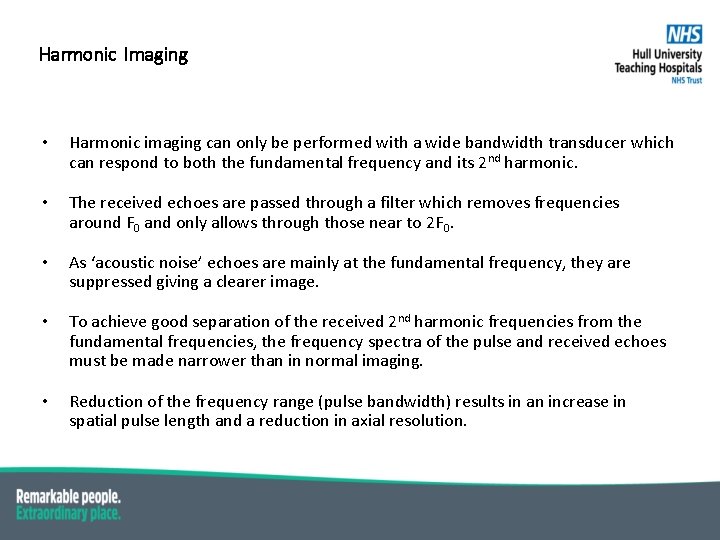
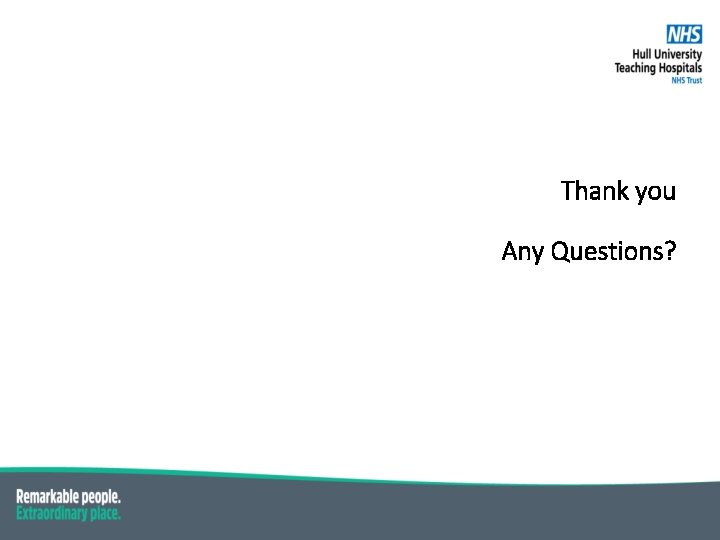
- Slides: 74
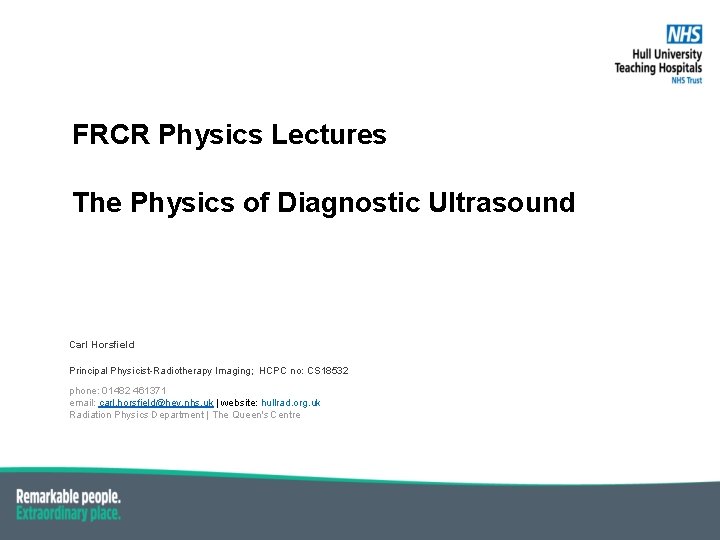
FRCR Physics Lectures The Physics of Diagnostic Ultrasound Carl Horsfield Principal Physicist-Radiotherapy Imaging; HCPC no: CS 18532 phone: 01482 461371 email: carl. horsfield@hey. nhs. uk | website: hullrad. org. uk Radiation Physics Department | The Queen's Centre
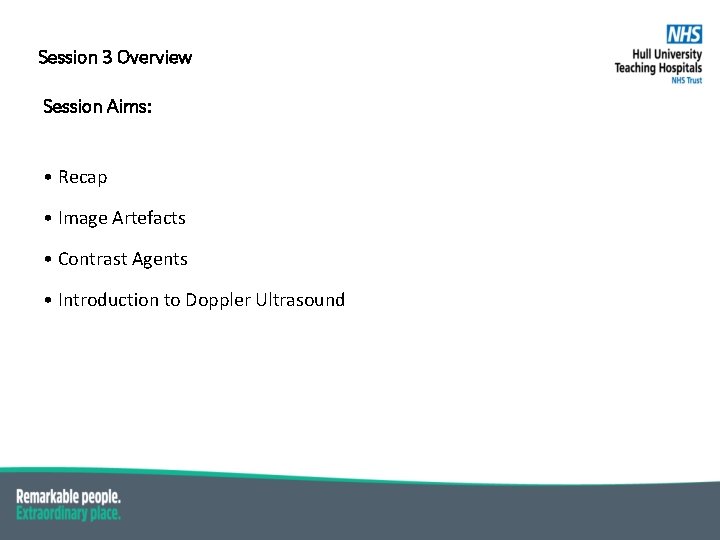
Session 3 Overview Session Aims: • Recap • Image Artefacts • Contrast Agents • Introduction to Doppler Ultrasound
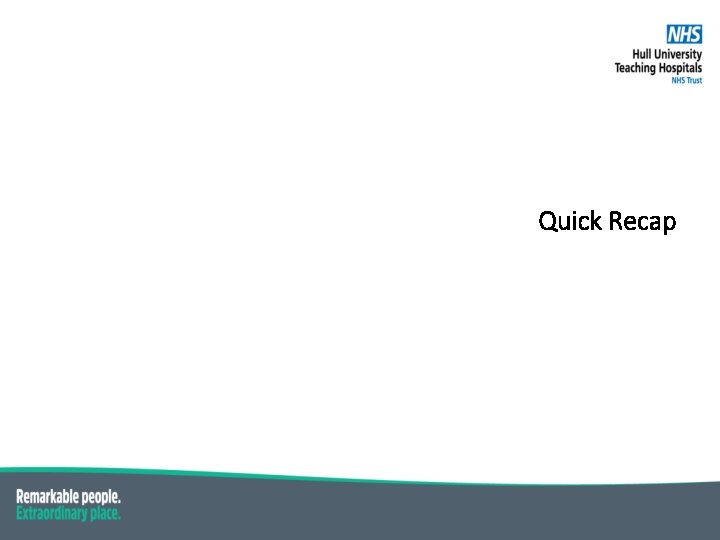
Quick Recap
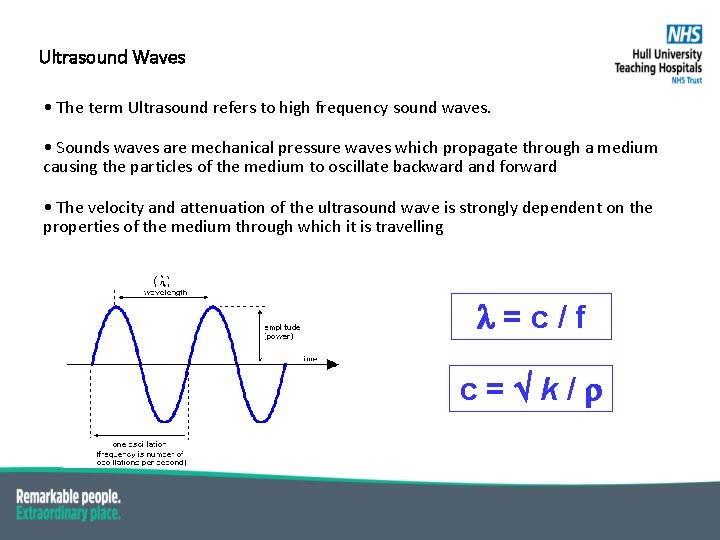
Ultrasound Waves • The term Ultrasound refers to high frequency sound waves. • Sounds waves are mechanical pressure waves which propagate through a medium causing the particles of the medium to oscillate backward and forward • The velocity and attenuation of the ultrasound wave is strongly dependent on the properties of the medium through which it is travelling =c/f c= k/
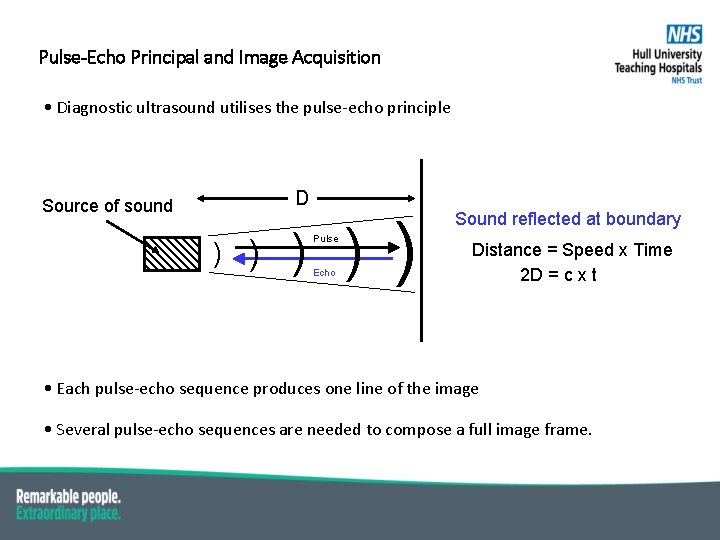
Pulse-Echo Principal and Image Acquisition • Diagnostic ultrasound utilises the pulse-echo principle D Source of sound ) ) ) Pulse Echo ) ) Sound reflected at boundary Distance = Speed x Time 2 D = c x t • Each pulse-echo sequence produces one line of the image • Several pulse-echo sequences are needed to compose a full image frame.
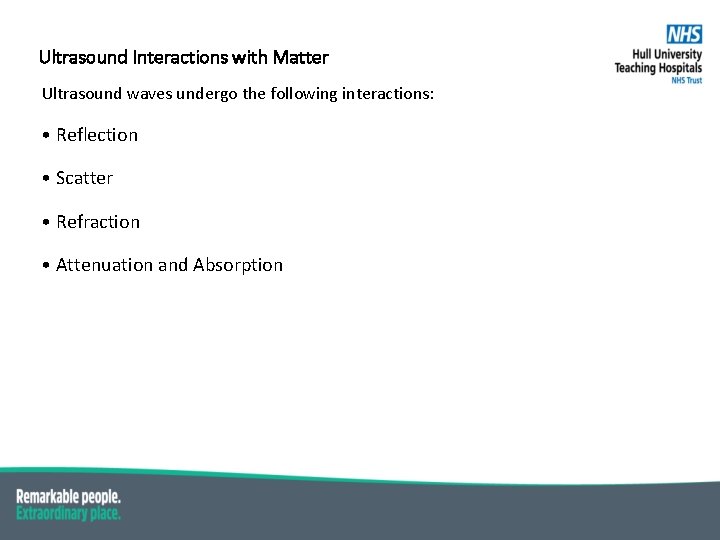
Ultrasound Interactions with Matter Ultrasound waves undergo the following interactions: • Reflection • Scatter • Refraction • Attenuation and Absorption
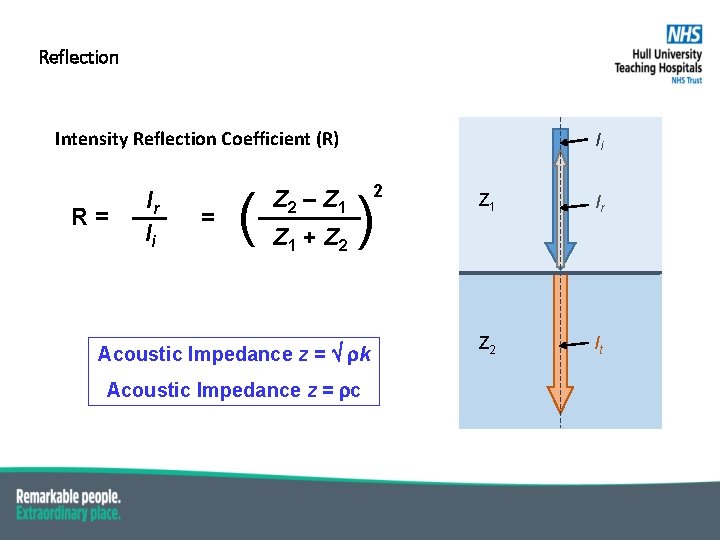
Reflection Intensity Reflection Coefficient (R) R= Ir Ii = ( Z 2 – Z 1 + Z 2 Ii 2 ) Acoustic Impedance z = k Acoustic Impedance z = c Z 1 Ir Z 2 It
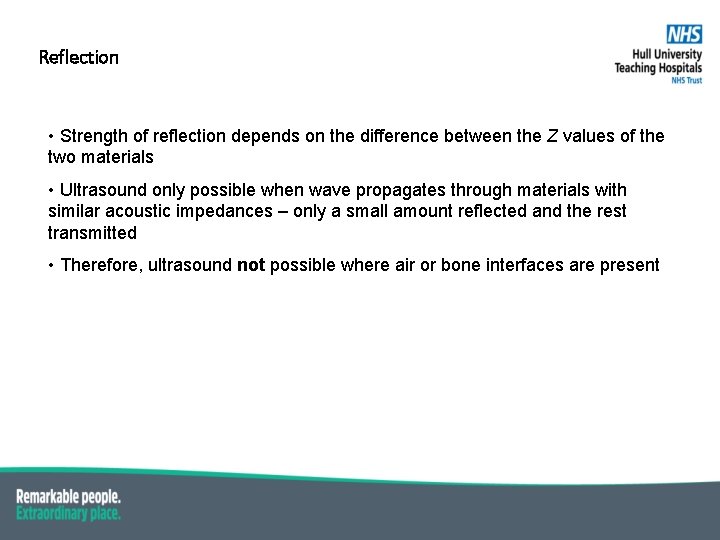
Reflection • Strength of reflection depends on the difference between the Z values of the two materials • Ultrasound only possible when wave propagates through materials with similar acoustic impedances – only a small amount reflected and the rest transmitted • Therefore, ultrasound not possible where air or bone interfaces are present
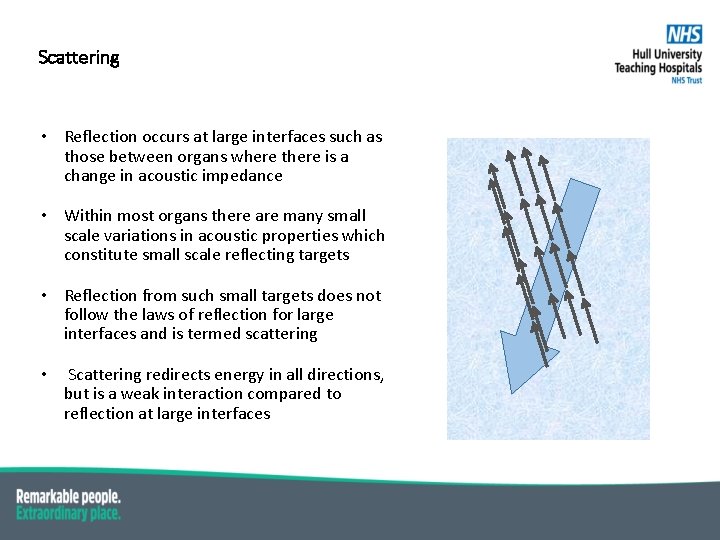
Scattering • Reflection occurs at large interfaces such as those between organs where there is a change in acoustic impedance • Within most organs there are many small scale variations in acoustic properties which constitute small scale reflecting targets • Reflection from such small targets does not follow the laws of reflection for large interfaces and is termed scattering • Scattering redirects energy in all directions, but is a weak interaction compared to reflection at large interfaces
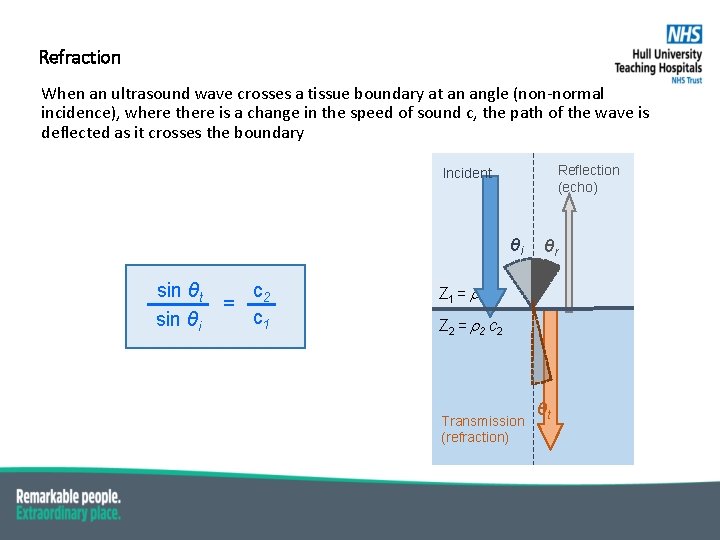
Refraction When an ultrasound wave crosses a tissue boundary at an angle (non-normal incidence), where there is a change in the speed of sound c, the path of the wave is deflected as it crosses the boundary Reflection (echo) Incident θi sin θt sin θi = c 2 c 1 θr Z 1 = 1 c 1 Z 2 = 2 c 2 Transmission (refraction) θt
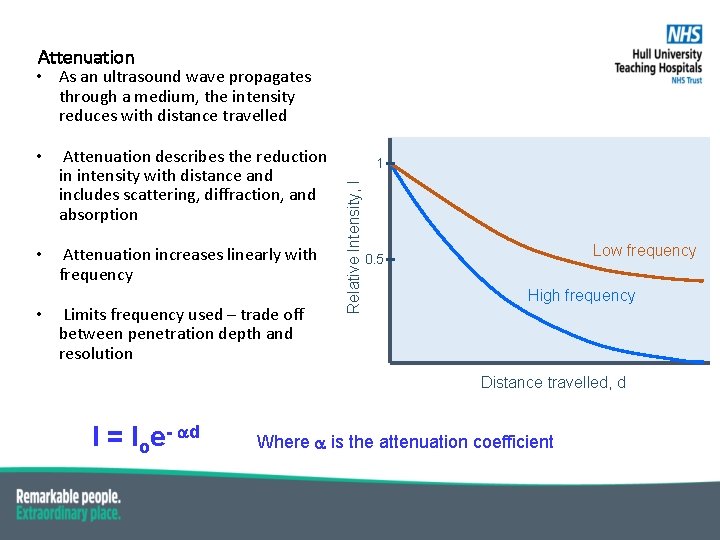
Attenuation • As an ultrasound wave propagates through a medium, the intensity reduces with distance travelled Attenuation describes the reduction in intensity with distance and includes scattering, diffraction, and absorption • Attenuation increases linearly with frequency • Limits frequency used – trade off between penetration depth and resolution 1 Relative Intensity, I • Low frequency 0. 5 High frequency Distance travelled, d I = Ioe- d Where is the attenuation coefficient
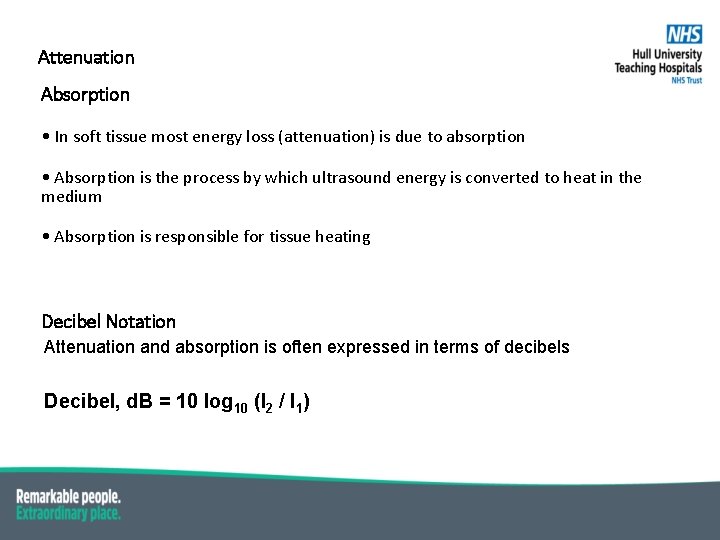
Attenuation Absorption • In soft tissue most energy loss (attenuation) is due to absorption • Absorption is the process by which ultrasound energy is converted to heat in the medium • Absorption is responsible for tissue heating Decibel Notation Attenuation and absorption is often expressed in terms of decibels Decibel, d. B = 10 log 10 (I 2 / I 1)
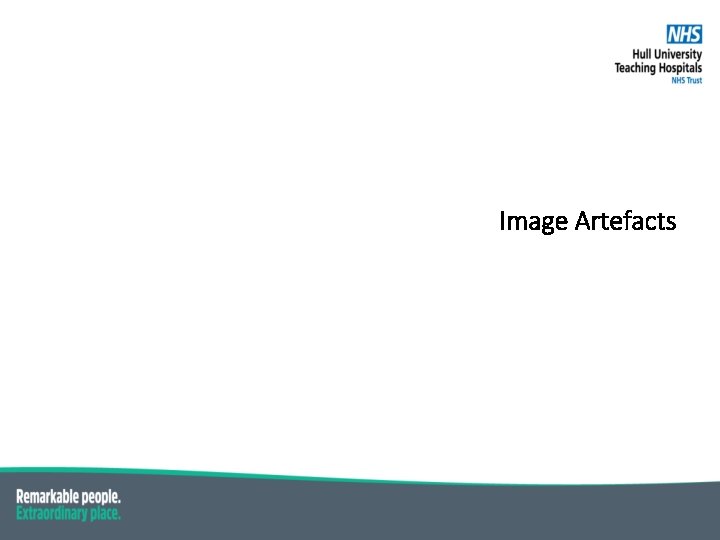
Image Artefacts
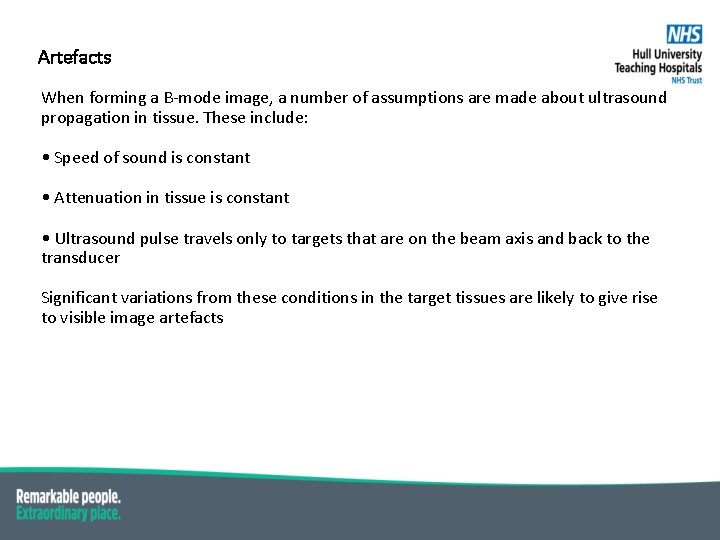
Artefacts When forming a B-mode image, a number of assumptions are made about ultrasound propagation in tissue. These include: • Speed of sound is constant • Attenuation in tissue is constant • Ultrasound pulse travels only to targets that are on the beam axis and back to the transducer Significant variations from these conditions in the target tissues are likely to give rise to visible image artefacts
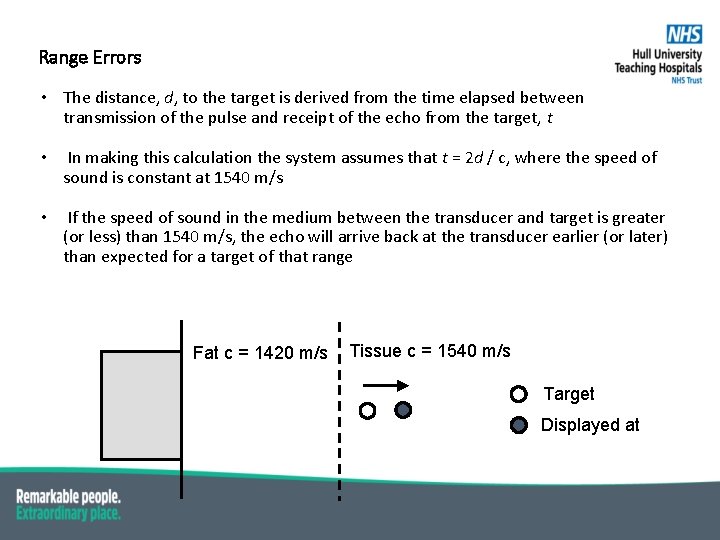
Range Errors • The distance, d, to the target is derived from the time elapsed between transmission of the pulse and receipt of the echo from the target, t • In making this calculation the system assumes that t = 2 d / c, where the speed of sound is constant at 1540 m/s • If the speed of sound in the medium between the transducer and target is greater (or less) than 1540 m/s, the echo will arrive back at the transducer earlier (or later) than expected for a target of that range Fat c = 1420 m/s Tissue c = 1540 m/s Target Displayed at
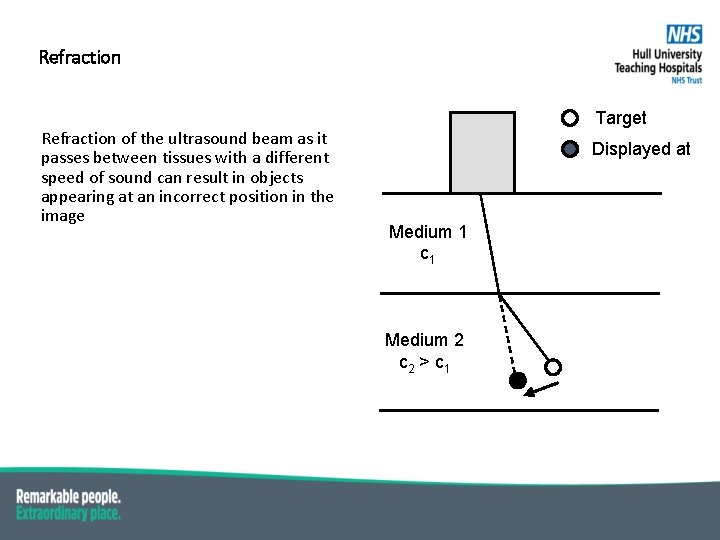
Refraction of the ultrasound beam as it passes between tissues with a different speed of sound can result in objects appearing at an incorrect position in the image Target Displayed at Medium 1 c 1 Medium 2 c 2 > c 1
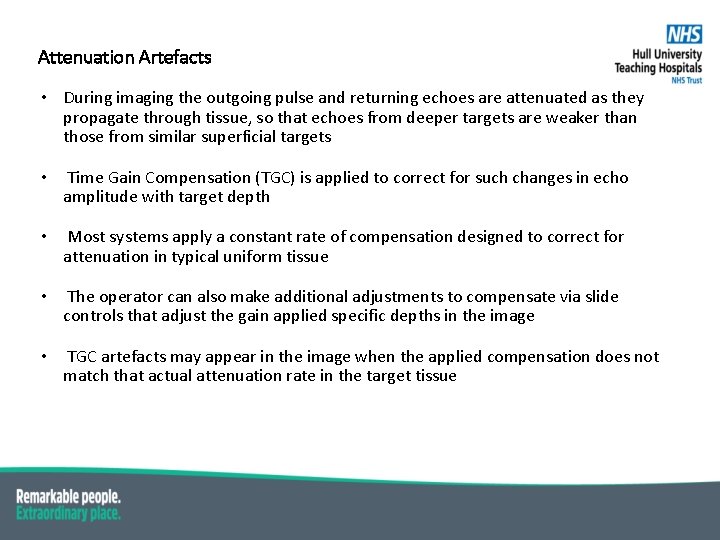
Attenuation Artefacts • During imaging the outgoing pulse and returning echoes are attenuated as they propagate through tissue, so that echoes from deeper targets are weaker than those from similar superficial targets • Time Gain Compensation (TGC) is applied to correct for such changes in echo amplitude with target depth • Most systems apply a constant rate of compensation designed to correct for attenuation in typical uniform tissue • The operator can also make additional adjustments to compensate via slide controls that adjust the gain applied specific depths in the image • TGC artefacts may appear in the image when the applied compensation does not match that actual attenuation rate in the target tissue
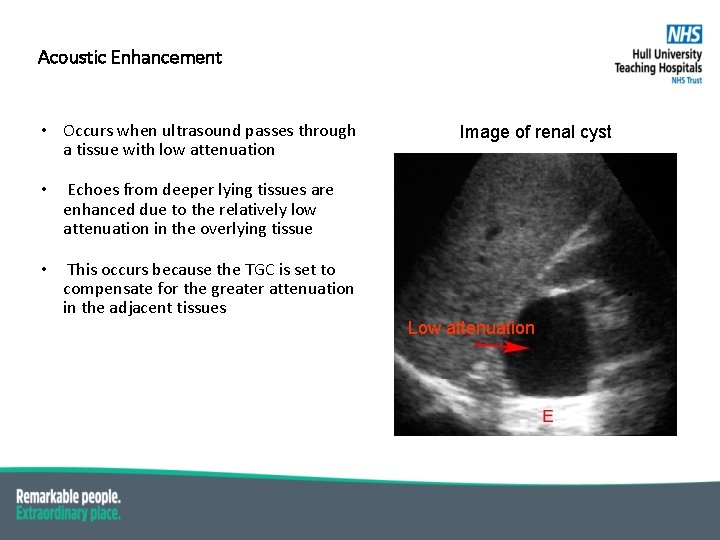
Acoustic Enhancement • Occurs when ultrasound passes through a tissue with low attenuation • Echoes from deeper lying tissues are enhanced due to the relatively low attenuation in the overlying tissue • This occurs because the TGC is set to compensate for the greater attenuation in the adjacent tissues Image of renal cyst Low attenuation
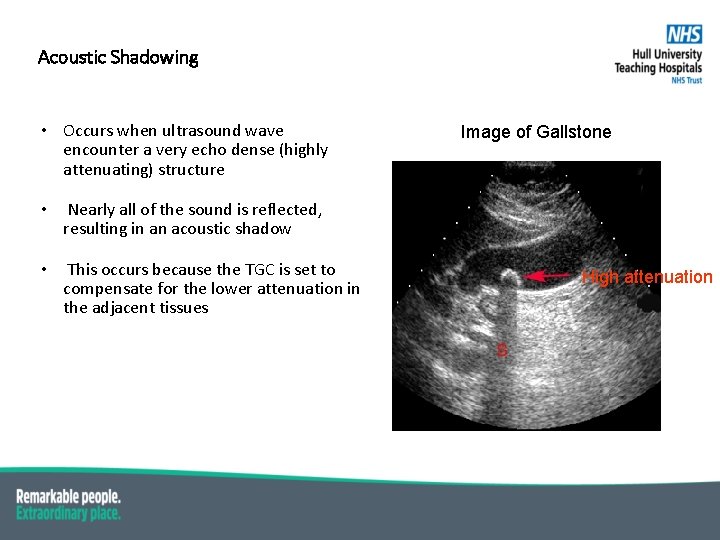
Acoustic Shadowing • Occurs when ultrasound wave encounter a very echo dense (highly attenuating) structure • Nearly all of the sound is reflected, resulting in an acoustic shadow • This occurs because the TGC is set to compensate for the lower attenuation in the adjacent tissues Image of Gallstone High attenuation
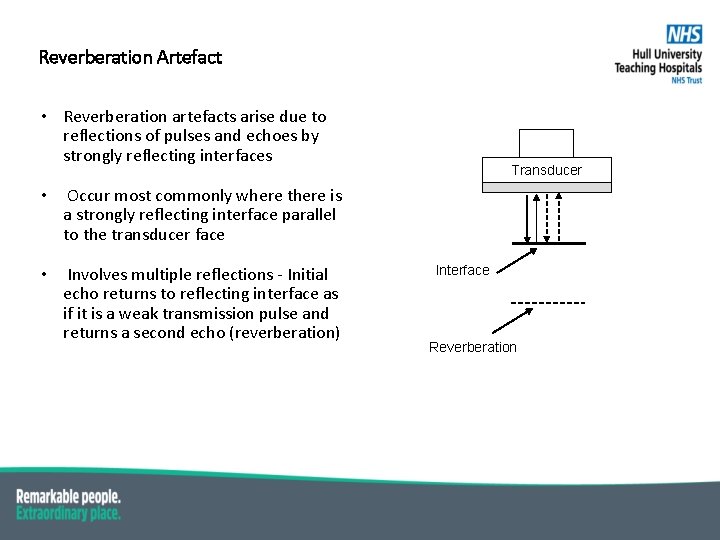
Reverberation Artefact • Reverberation artefacts arise due to reflections of pulses and echoes by strongly reflecting interfaces • Occur most commonly where there is a strongly reflecting interface parallel to the transducer face • Involves multiple reflections - Initial echo returns to reflecting interface as if it is a weak transmission pulse and returns a second echo (reverberation) Transducer Interface Reverberation
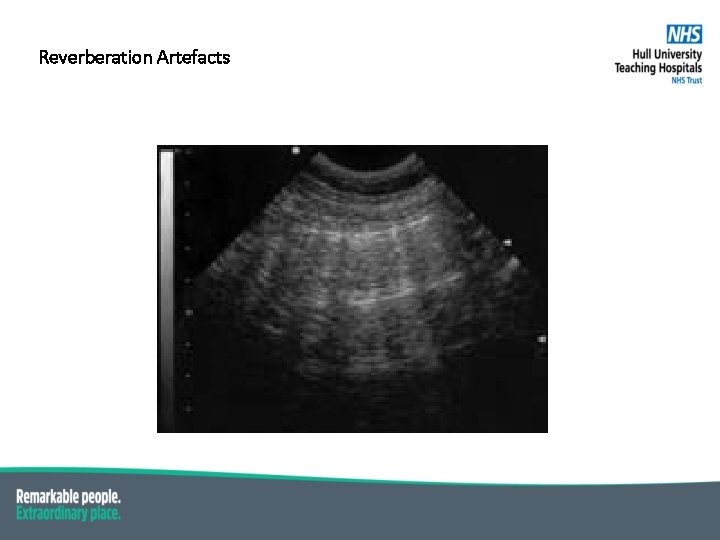
Reverberation Artefacts
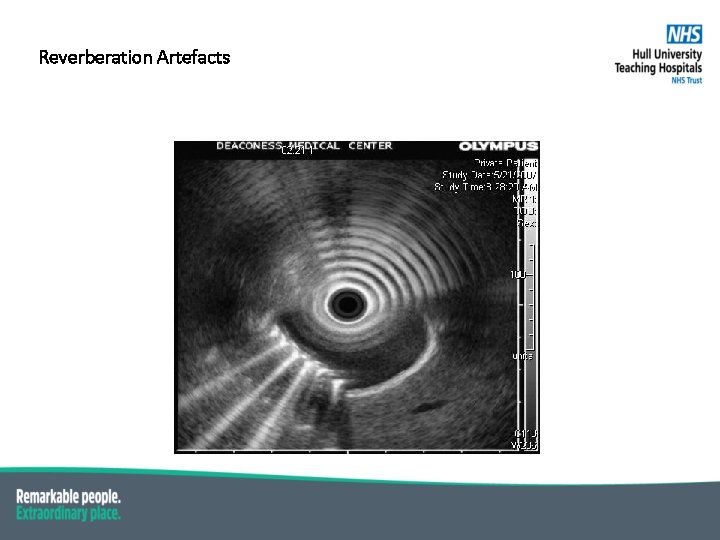
Reverberation Artefacts
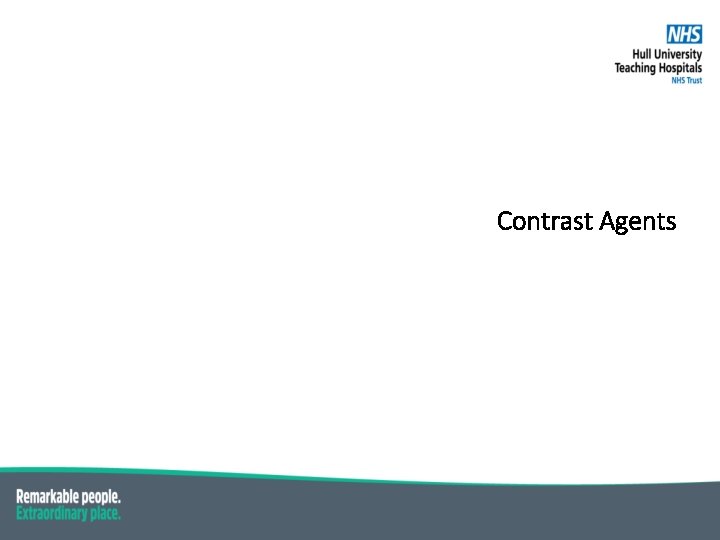
Contrast Agents
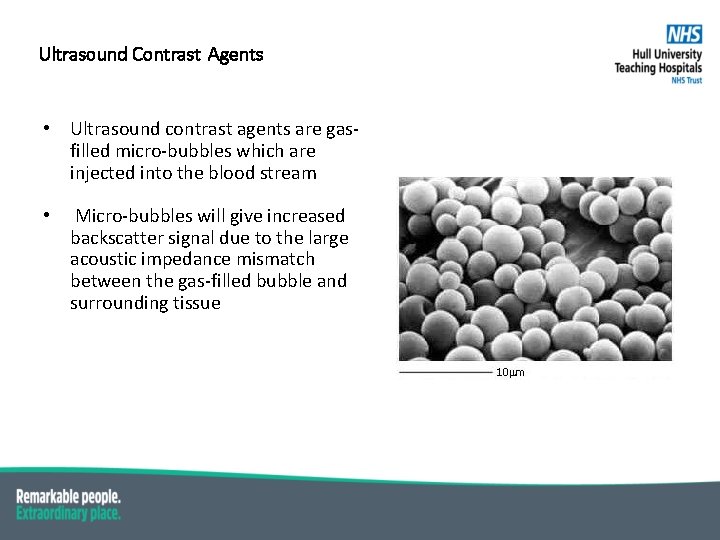
Ultrasound Contrast Agents • Ultrasound contrast agents are gasfilled micro-bubbles which are injected into the blood stream • Micro-bubbles will give increased backscatter signal due to the large acoustic impedance mismatch between the gas-filled bubble and surrounding tissue
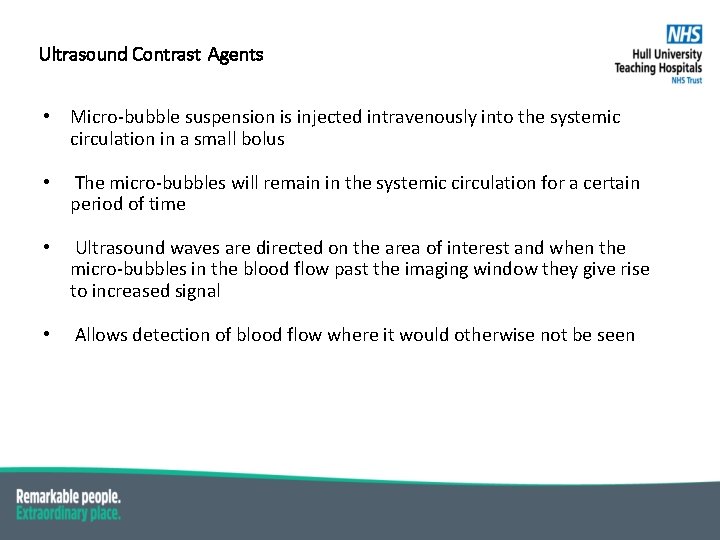
Ultrasound Contrast Agents • Micro-bubble suspension is injected intravenously into the systemic circulation in a small bolus • The micro-bubbles will remain in the systemic circulation for a certain period of time • Ultrasound waves are directed on the area of interest and when the micro-bubbles in the blood flow past the imaging window they give rise to increased signal • Allows detection of blood flow where it would otherwise not be seen
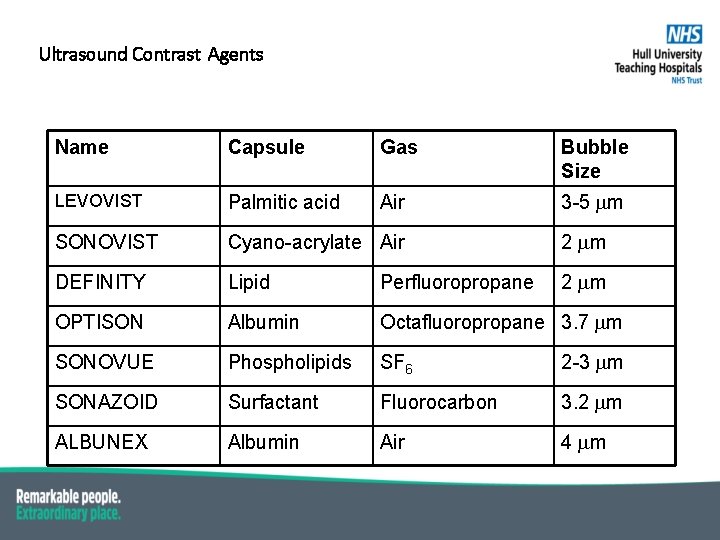
Ultrasound Contrast Agents Name Capsule Gas Bubble Size LEVOVIST Palmitic acid Air 3 -5 m SONOVIST Cyano-acrylate Air 2 m DEFINITY Lipid Perfluoropropane 2 m OPTISON Albumin Octafluoropropane 3. 7 m SONOVUE Phospholipids SF 6 2 -3 m SONAZOID Surfactant Fluorocarbon 3. 2 m ALBUNEX Albumin Air 4 m
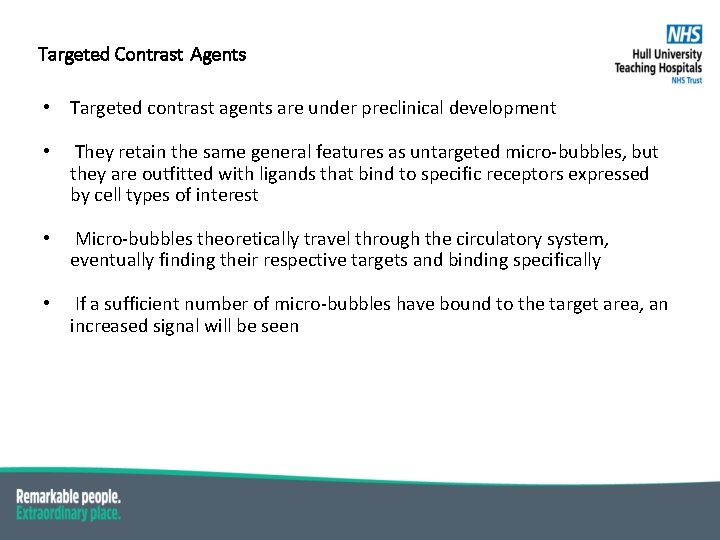
Targeted Contrast Agents • Targeted contrast agents are under preclinical development • They retain the same general features as untargeted micro-bubbles, but they are outfitted with ligands that bind to specific receptors expressed by cell types of interest • Micro-bubbles theoretically travel through the circulatory system, eventually finding their respective targets and binding specifically • If a sufficient number of micro-bubbles have bound to the target area, an increased signal will be seen
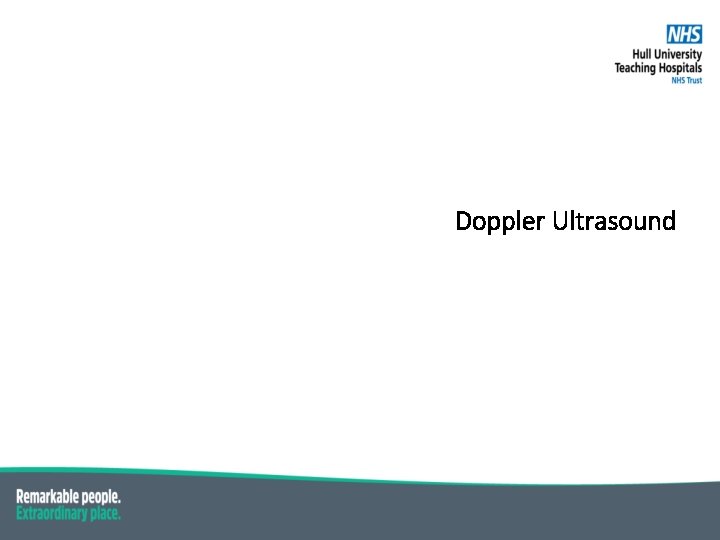
Doppler Ultrasound
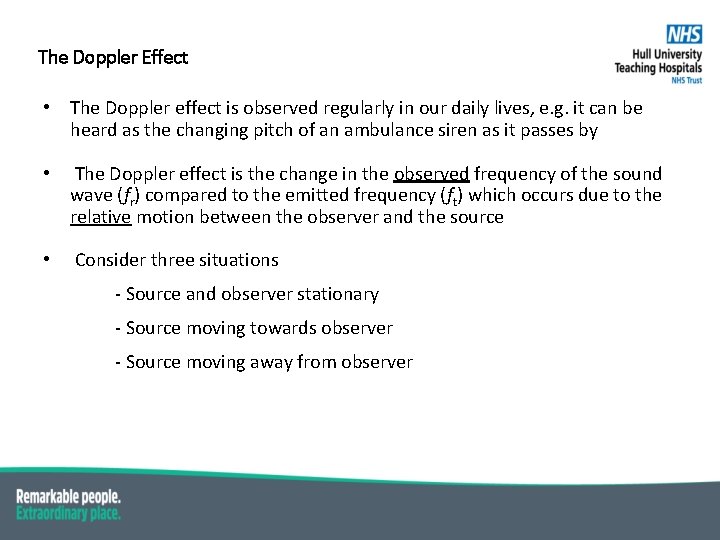
The Doppler Effect • The Doppler effect is observed regularly in our daily lives, e. g. it can be heard as the changing pitch of an ambulance siren as it passes by • The Doppler effect is the change in the observed frequency of the sound wave (fr) compared to the emitted frequency (ft) which occurs due to the relative motion between the observer and the source • Consider three situations - Source and observer stationary - Source moving towards observer - Source moving away from observer
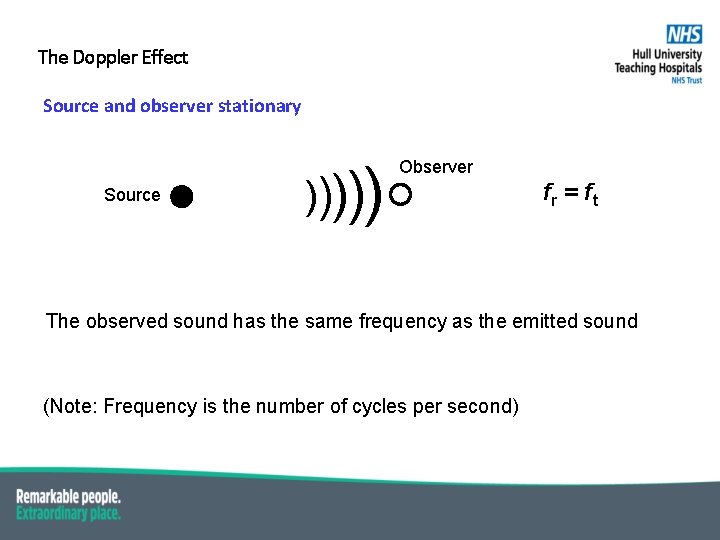
The Doppler Effect Source and observer stationary Source ) )))) Observer fr = f t The observed sound has the same frequency as the emitted sound (Note: Frequency is the number of cycles per second)
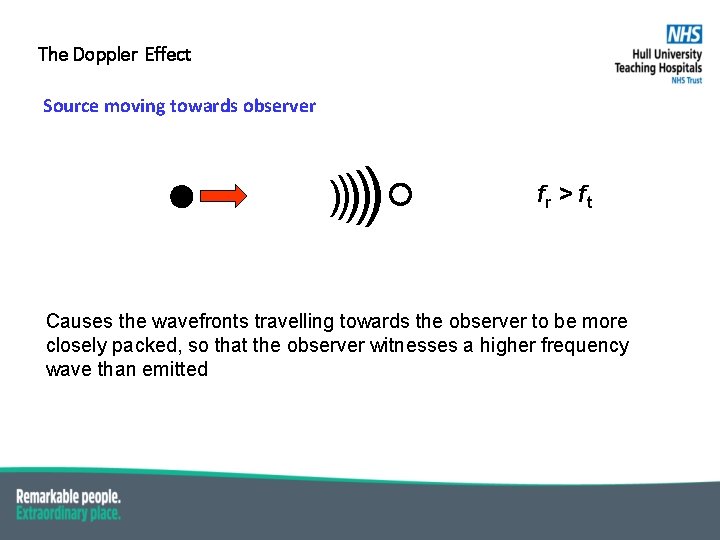
The Doppler Effect Source moving towards observer ) )))) fr > f t Causes the wavefronts travelling towards the observer to be more closely packed, so that the observer witnesses a higher frequency wave than emitted
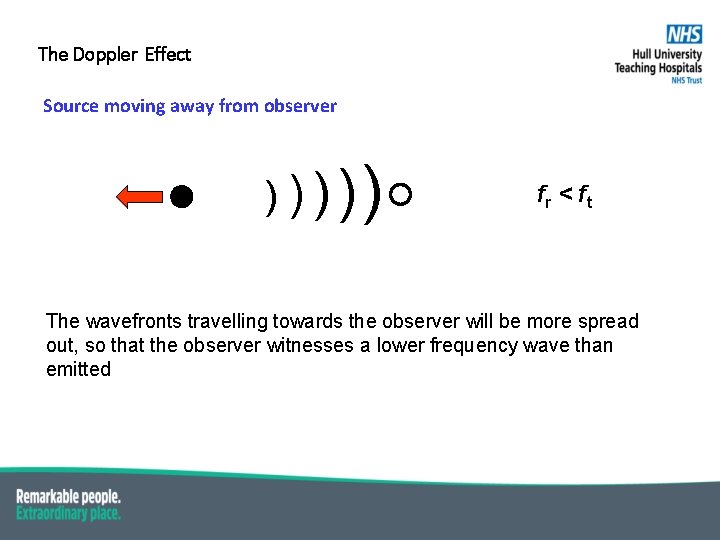
The Doppler Effect Source moving away from observer ) ))) ) fr < f t The wavefronts travelling towards the observer will be more spread out, so that the observer witnesses a lower frequency wave than emitted
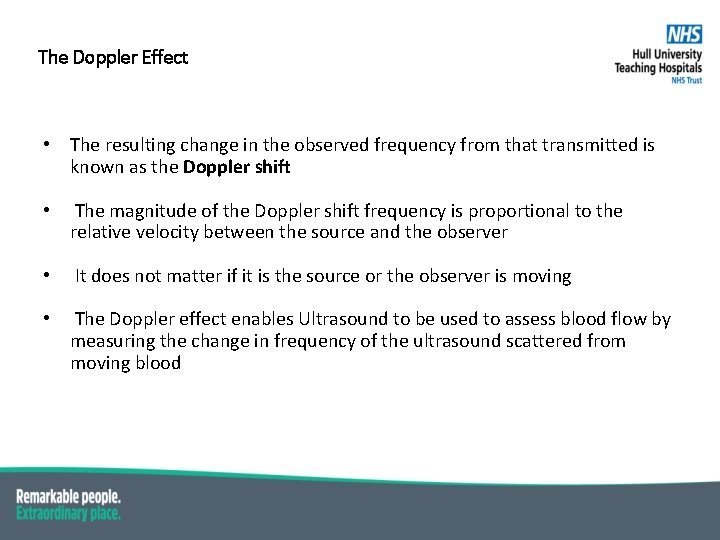
The Doppler Effect • The resulting change in the observed frequency from that transmitted is known as the Doppler shift • The magnitude of the Doppler shift frequency is proportional to the relative velocity between the source and the observer • It does not matter if it is the source or the observer is moving • The Doppler effect enables Ultrasound to be used to assess blood flow by measuring the change in frequency of the ultrasound scattered from moving blood
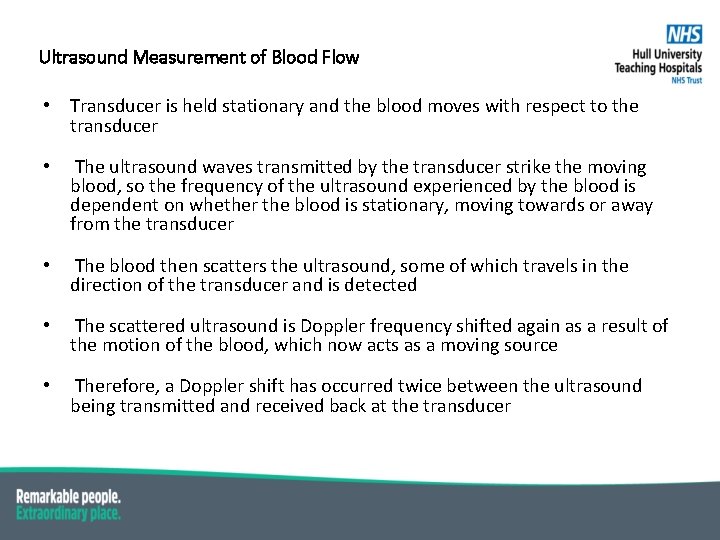
Ultrasound Measurement of Blood Flow • Transducer is held stationary and the blood moves with respect to the transducer • The ultrasound waves transmitted by the transducer strike the moving blood, so the frequency of the ultrasound experienced by the blood is dependent on whether the blood is stationary, moving towards or away from the transducer • The blood then scatters the ultrasound, some of which travels in the direction of the transducer and is detected • The scattered ultrasound is Doppler frequency shifted again as a result of the motion of the blood, which now acts as a moving source • Therefore, a Doppler shift has occurred twice between the ultrasound being transmitted and received back at the transducer
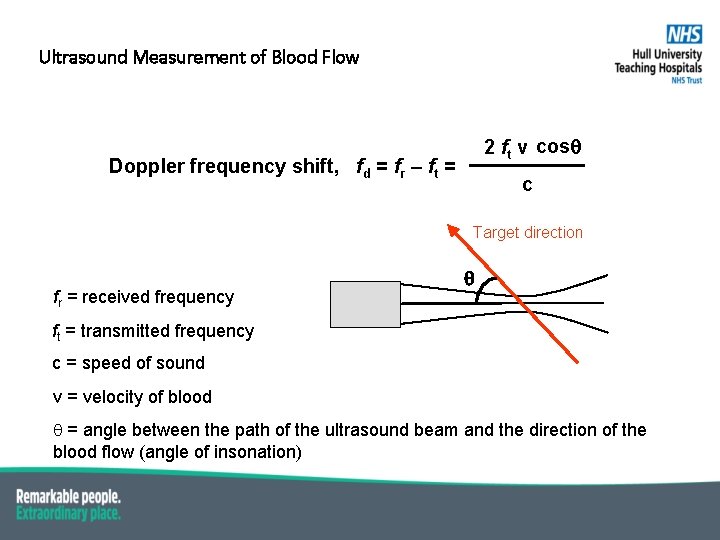
Ultrasound Measurement of Blood Flow 2 ft v cos Doppler frequency shift, fd = fr – ft = c Target direction fr = received frequency ft = transmitted frequency c = speed of sound v = velocity of blood = angle between the path of the ultrasound beam and the direction of the blood flow (angle of insonation)
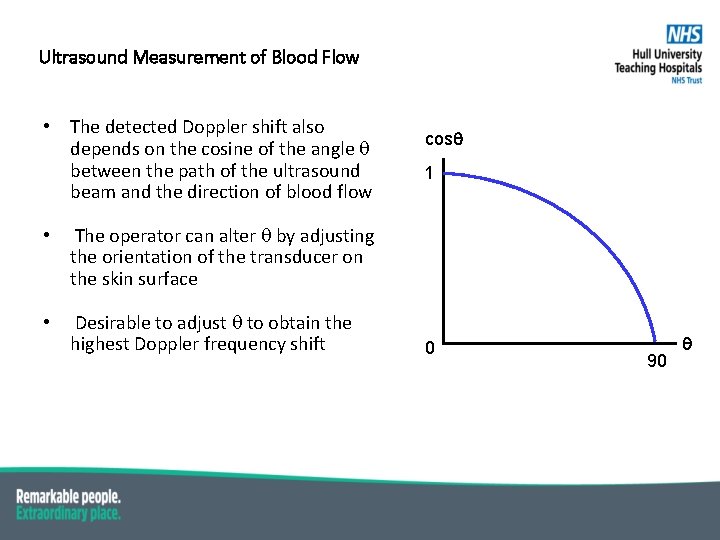
Ultrasound Measurement of Blood Flow • The detected Doppler shift also depends on the cosine of the angle between the path of the ultrasound beam and the direction of blood flow • The operator can alter by adjusting the orientation of the transducer on the skin surface • Desirable to adjust to obtain the highest Doppler frequency shift cos 1 0 90
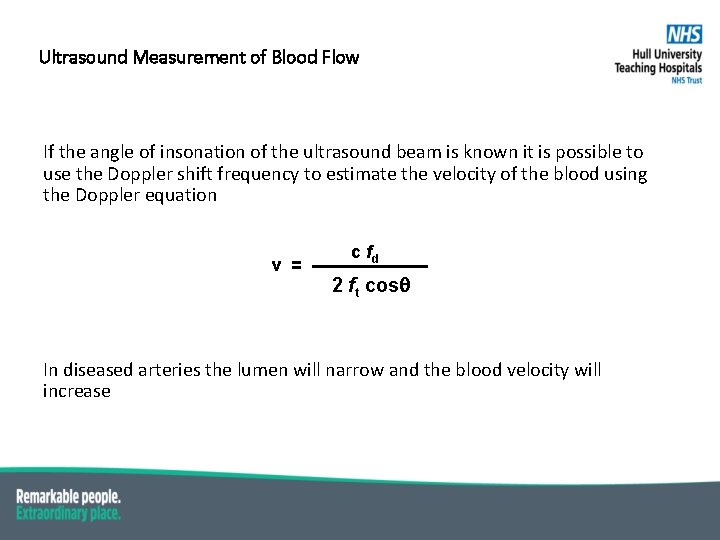
Ultrasound Measurement of Blood Flow If the angle of insonation of the ultrasound beam is known it is possible to use the Doppler shift frequency to estimate the velocity of the blood using the Doppler equation v = c fd 2 ft cos In diseased arteries the lumen will narrow and the blood velocity will increase
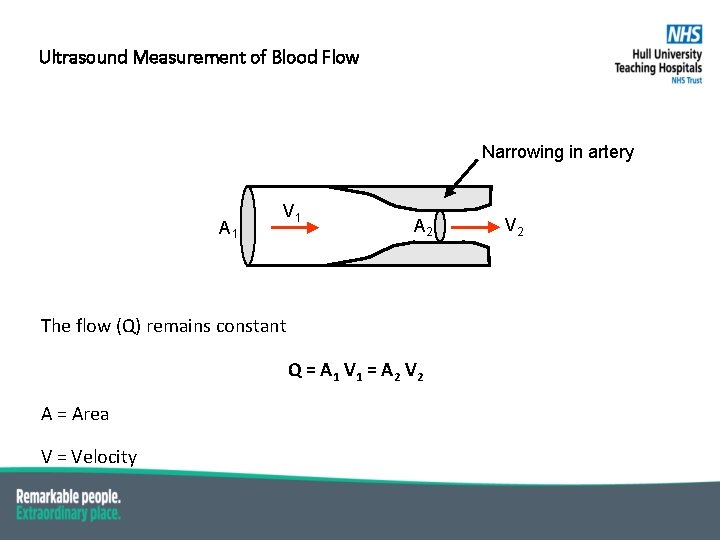
Ultrasound Measurement of Blood Flow Narrowing in artery A 1 V 1 A 2 The flow (Q) remains constant Q = A 1 V 1 = A 2 V 2 A = Area V = Velocity V 2
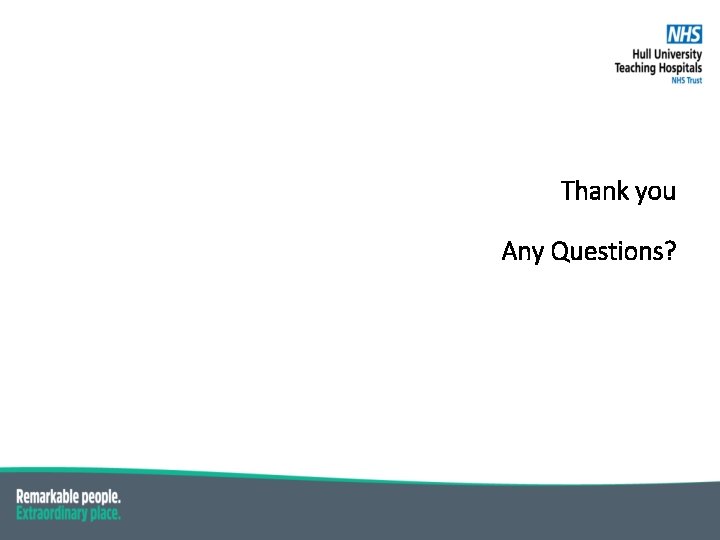
Thank you Any Questions?
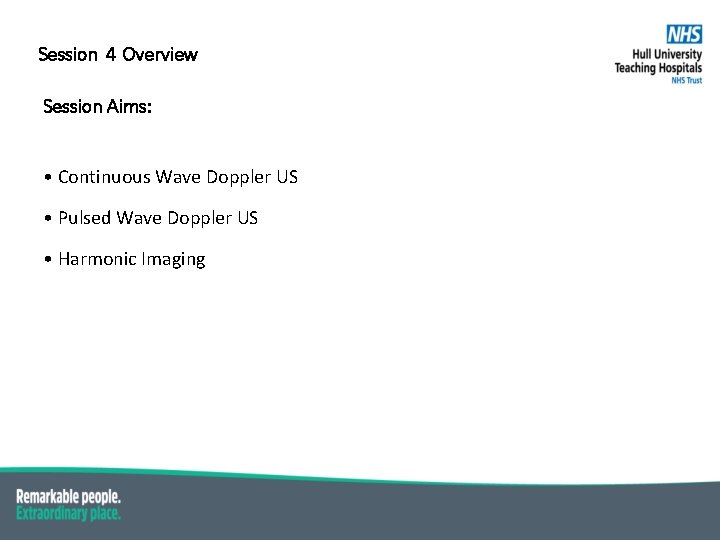
Session 4 Overview Session Aims: • Continuous Wave Doppler US • Pulsed Wave Doppler US • Harmonic Imaging
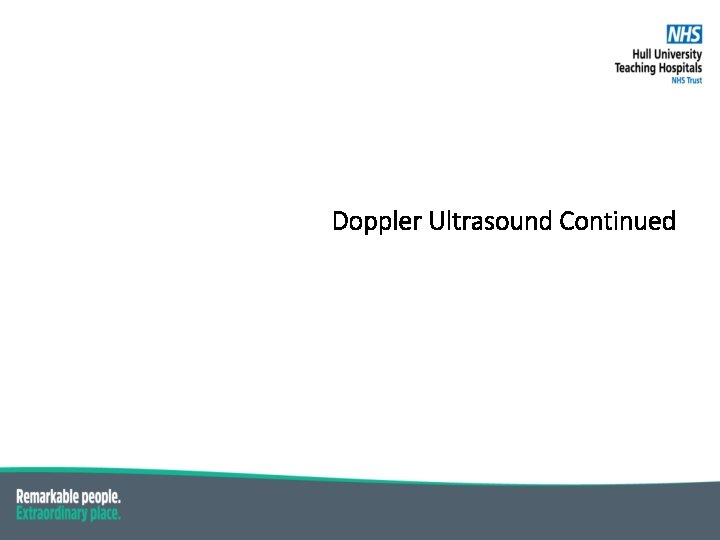
Doppler Ultrasound Continued
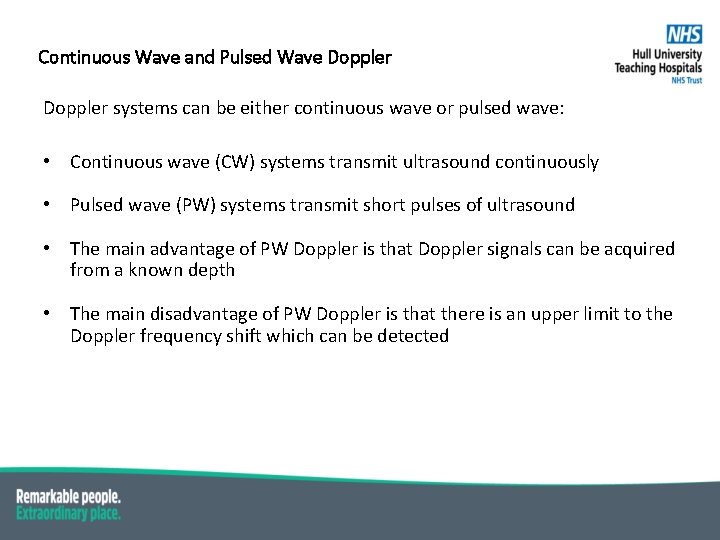
Continuous Wave and Pulsed Wave Doppler systems can be either continuous wave or pulsed wave: • Continuous wave (CW) systems transmit ultrasound continuously • Pulsed wave (PW) systems transmit short pulses of ultrasound • The main advantage of PW Doppler is that Doppler signals can be acquired from a known depth • The main disadvantage of PW Doppler is that there is an upper limit to the Doppler frequency shift which can be detected
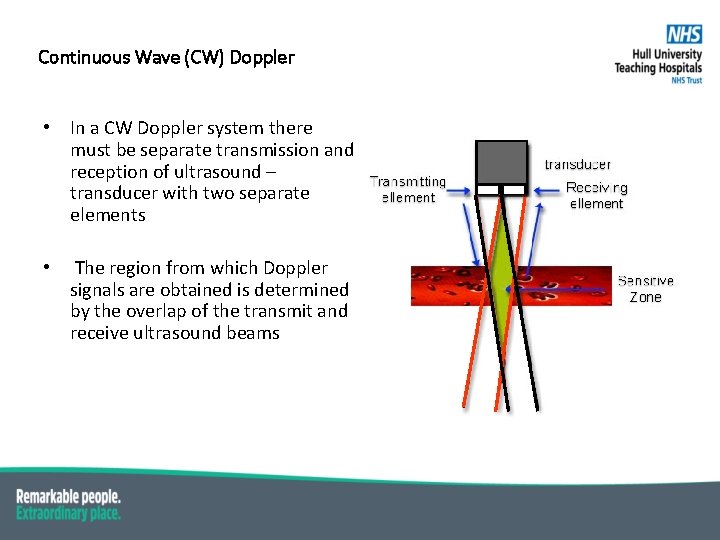
Continuous Wave (CW) Doppler • In a CW Doppler system there must be separate transmission and reception of ultrasound – transducer with two separate elements • The region from which Doppler signals are obtained is determined by the overlap of the transmit and receive ultrasound beams
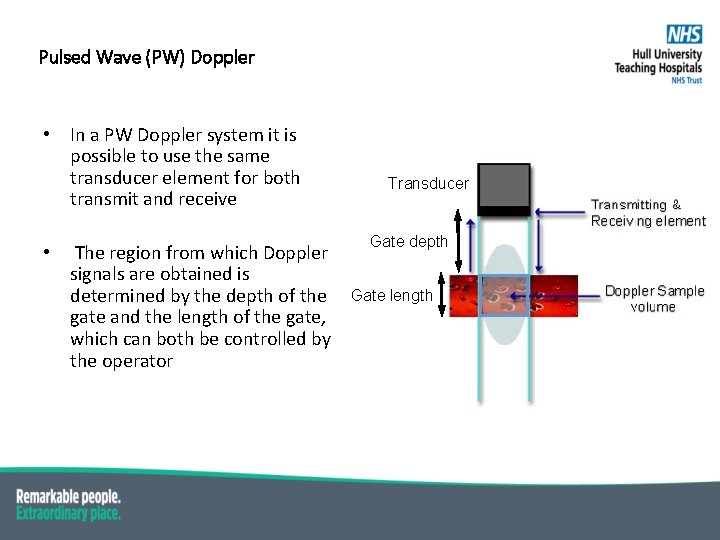
Pulsed Wave (PW) Doppler • In a PW Doppler system it is possible to use the same transducer element for both transmit and receive • The region from which Doppler signals are obtained is determined by the depth of the gate and the length of the gate, which can both be controlled by the operator Transducer Gate depth Gate length
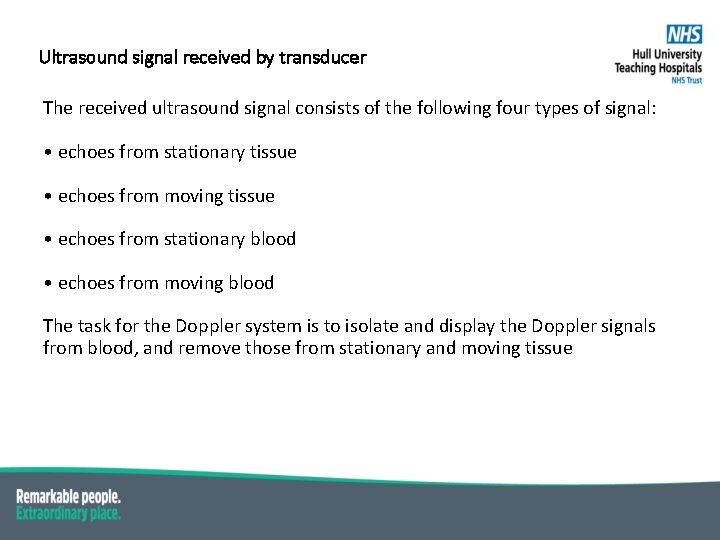
Ultrasound signal received by transducer The received ultrasound signal consists of the following four types of signal: • echoes from stationary tissue • echoes from moving tissue • echoes from stationary blood • echoes from moving blood The task for the Doppler system is to isolate and display the Doppler signals from blood, and remove those from stationary and moving tissue
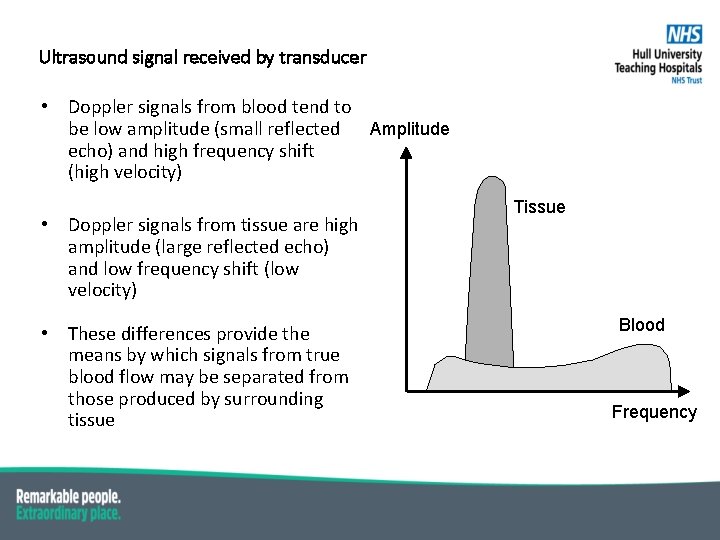
Ultrasound signal received by transducer • Doppler signals from blood tend to be low amplitude (small reflected Amplitude echo) and high frequency shift (high velocity) • Doppler signals from tissue are high amplitude (large reflected echo) and low frequency shift (low velocity) • These differences provide the means by which signals from true blood flow may be separated from those produced by surrounding tissue Tissue Blood Frequency
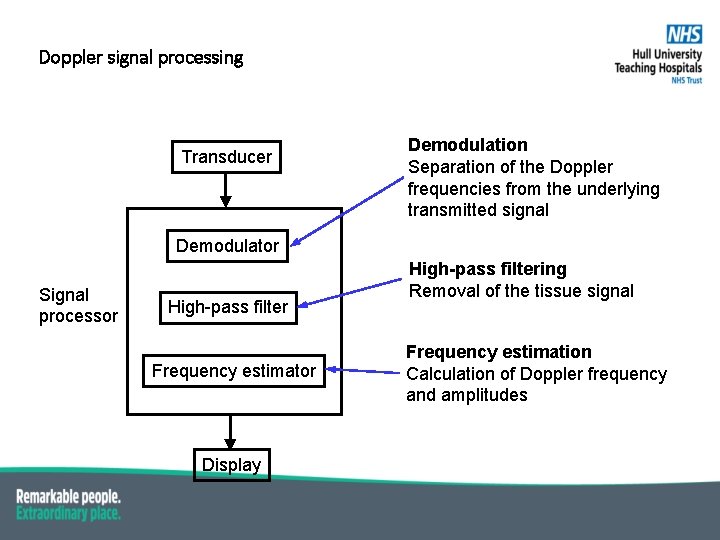
Doppler signal processing Transducer Demodulation Separation of the Doppler frequencies from the underlying transmitted signal Demodulator Signal processor High-pass filter Frequency estimator Display High-pass filtering Removal of the tissue signal Frequency estimation Calculation of Doppler frequency and amplitudes
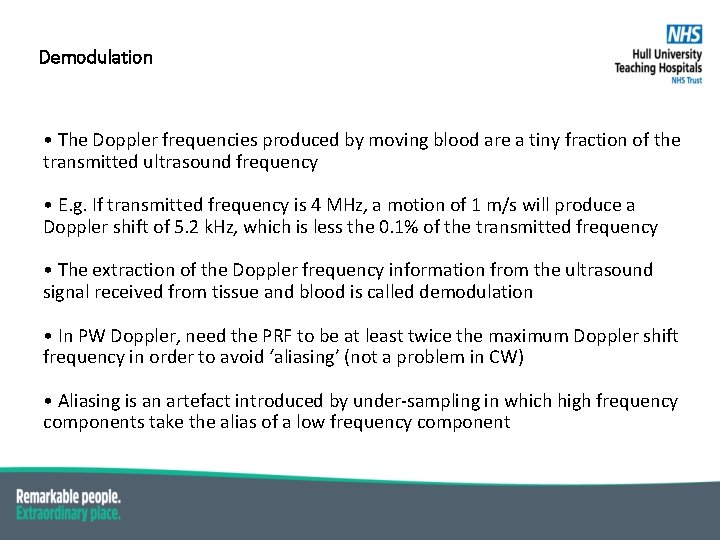
Demodulation • The Doppler frequencies produced by moving blood are a tiny fraction of the transmitted ultrasound frequency • E. g. If transmitted frequency is 4 MHz, a motion of 1 m/s will produce a Doppler shift of 5. 2 k. Hz, which is less the 0. 1% of the transmitted frequency • The extraction of the Doppler frequency information from the ultrasound signal received from tissue and blood is called demodulation • In PW Doppler, need the PRF to be at least twice the maximum Doppler shift frequency in order to avoid ‘aliasing’ (not a problem in CW) • Aliasing is an artefact introduced by under-sampling in which high frequency components take the alias of a low frequency component
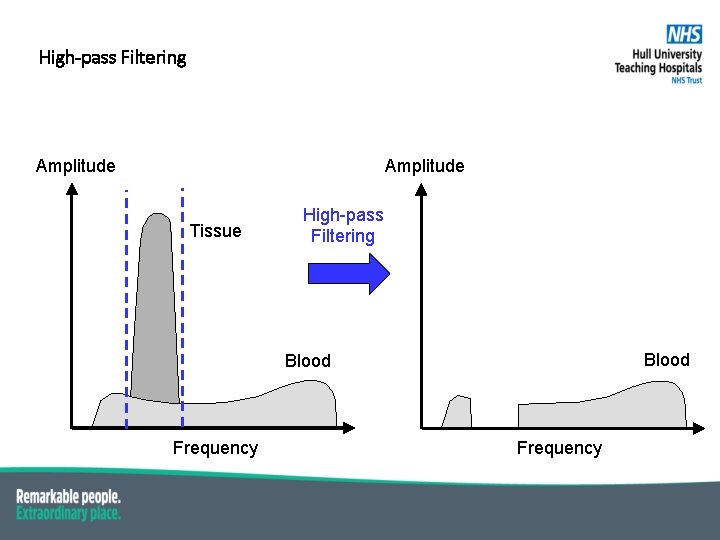
High-pass Filtering Amplitude Tissue High-pass Filtering Blood Frequency
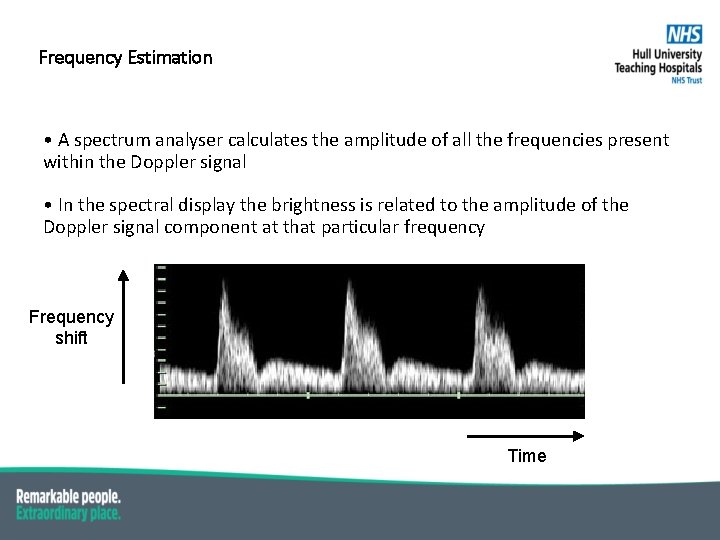
Frequency Estimation • A spectrum analyser calculates the amplitude of all the frequencies present within the Doppler signal • In the spectral display the brightness is related to the amplitude of the Doppler signal component at that particular frequency Frequency shift Time
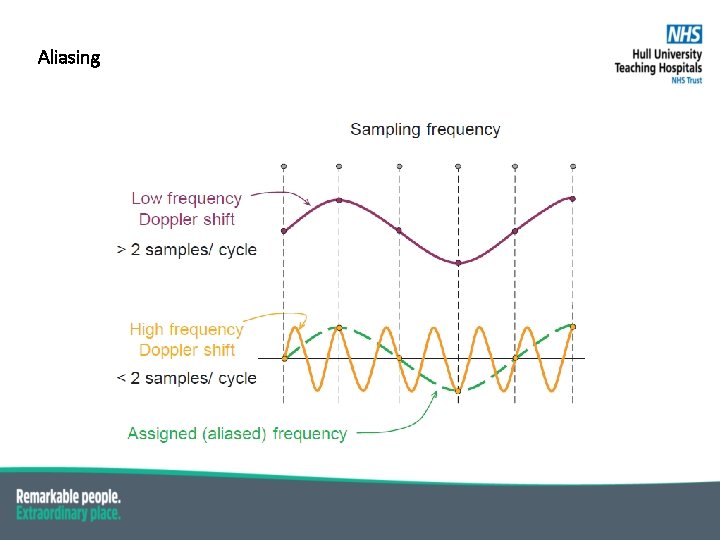
Aliasing
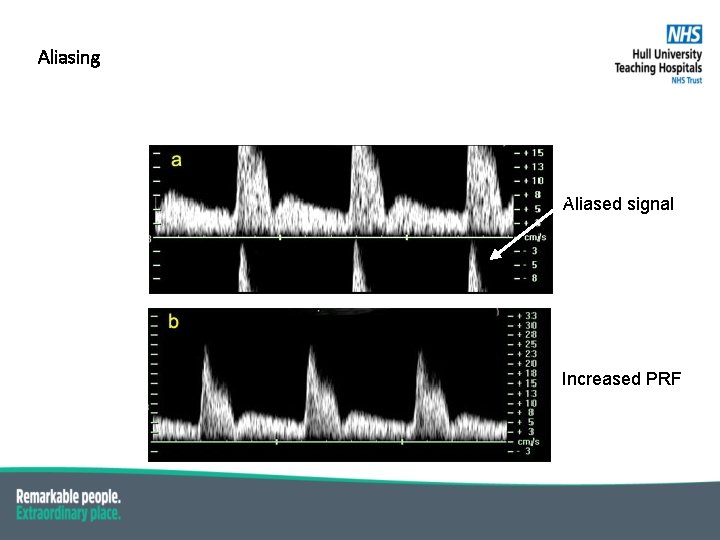
Aliasing Aliased signal Increased PRF
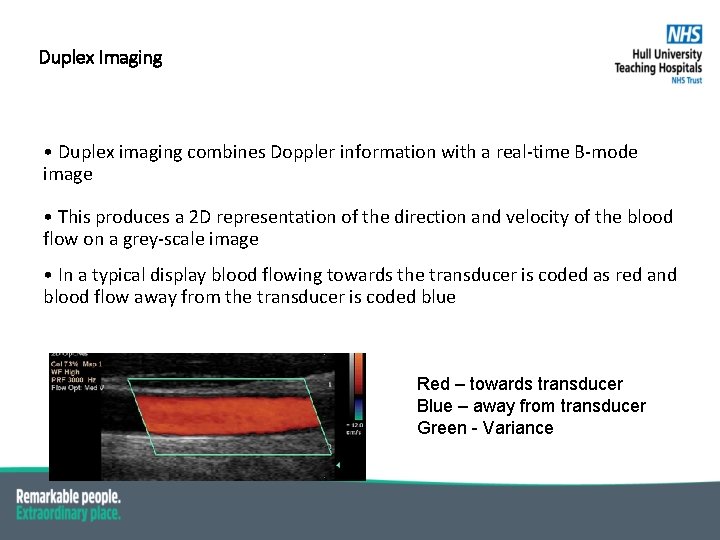
Duplex Imaging • Duplex imaging combines Doppler information with a real-time B-mode image • This produces a 2 D representation of the direction and velocity of the blood flow on a grey-scale image • In a typical display blood flowing towards the transducer is coded as red and blood flow away from the transducer is coded blue Red – towards transducer Blue – away from transducer Green - Variance
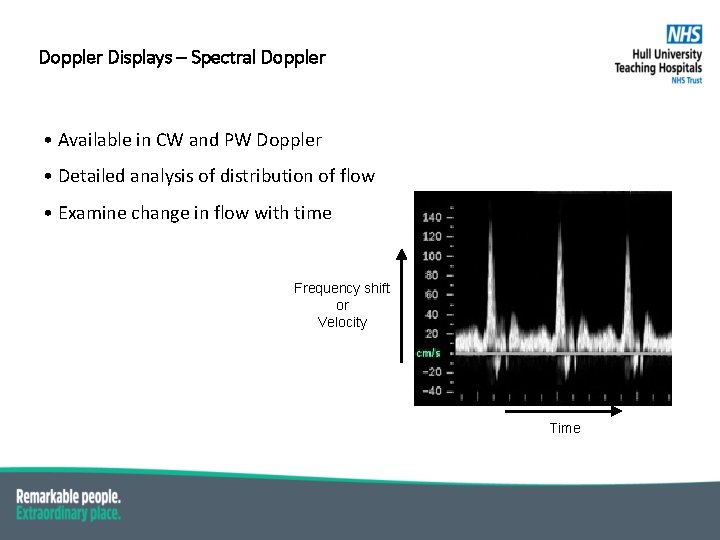
Doppler Displays – Spectral Doppler • Available in CW and PW Doppler • Detailed analysis of distribution of flow • Examine change in flow with time Frequency shift or Velocity Time
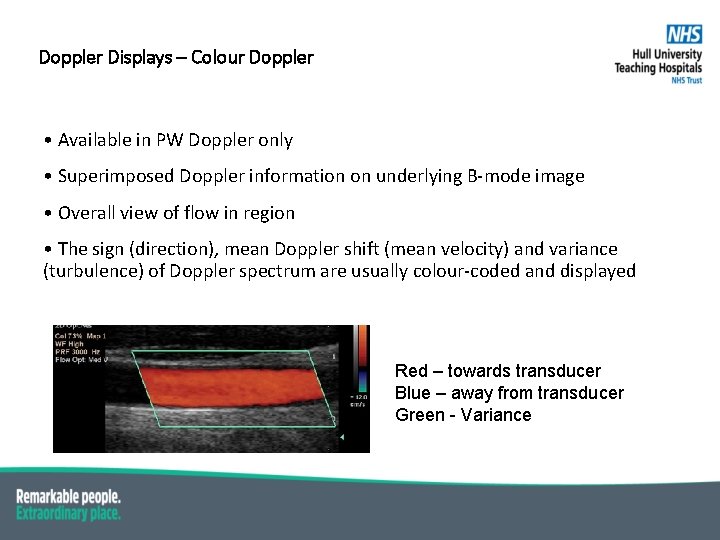
Doppler Displays – Colour Doppler • Available in PW Doppler only • Superimposed Doppler information on underlying B-mode image • Overall view of flow in region • The sign (direction), mean Doppler shift (mean velocity) and variance (turbulence) of Doppler spectrum are usually colour-coded and displayed Red – towards transducer Blue – away from transducer Green - Variance
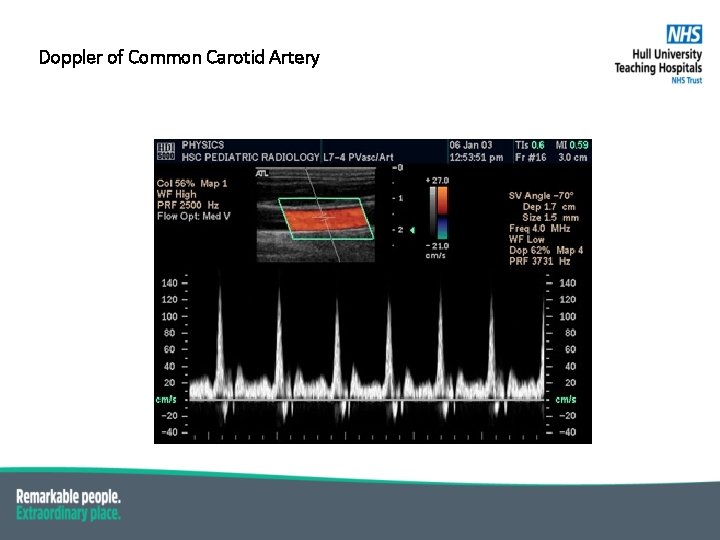
Doppler of Common Carotid Artery
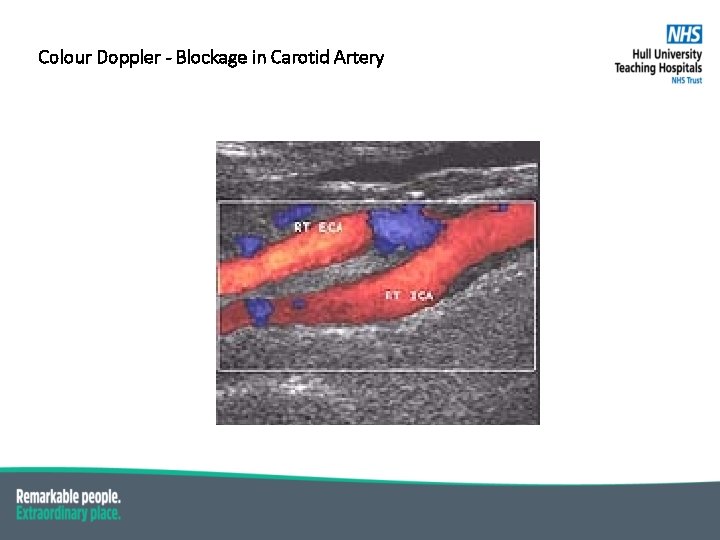
Colour Doppler - Blockage in Carotid Artery
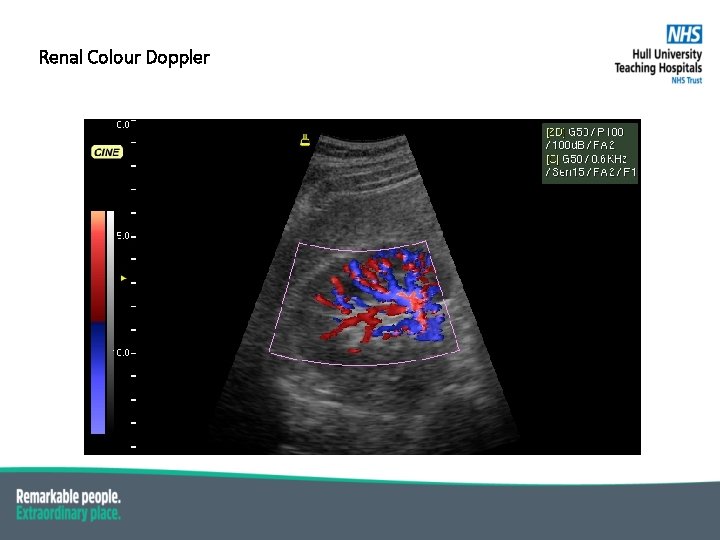
Renal Colour Doppler
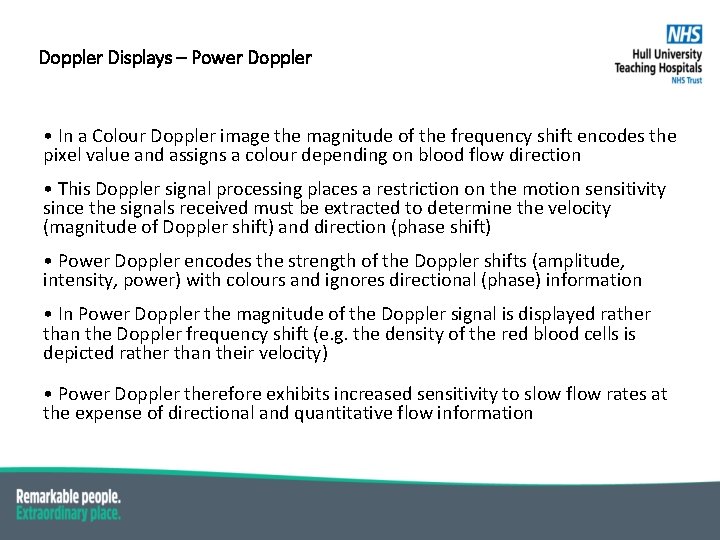
Doppler Displays – Power Doppler • In a Colour Doppler image the magnitude of the frequency shift encodes the pixel value and assigns a colour depending on blood flow direction • This Doppler signal processing places a restriction on the motion sensitivity since the signals received must be extracted to determine the velocity (magnitude of Doppler shift) and direction (phase shift) • Power Doppler encodes the strength of the Doppler shifts (amplitude, intensity, power) with colours and ignores directional (phase) information • In Power Doppler the magnitude of the Doppler signal is displayed rather than the Doppler frequency shift (e. g. the density of the red blood cells is depicted rather than their velocity) • Power Doppler therefore exhibits increased sensitivity to slow flow rates at the expense of directional and quantitative flow information
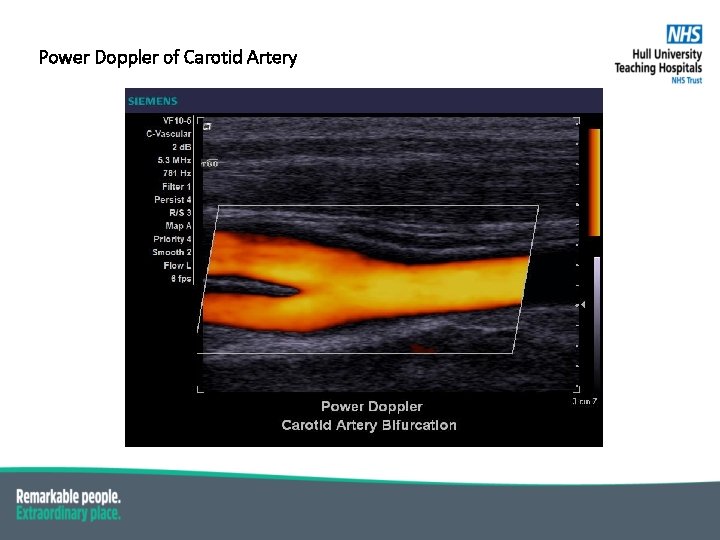
Power Doppler of Carotid Artery Suspicious dark lesion
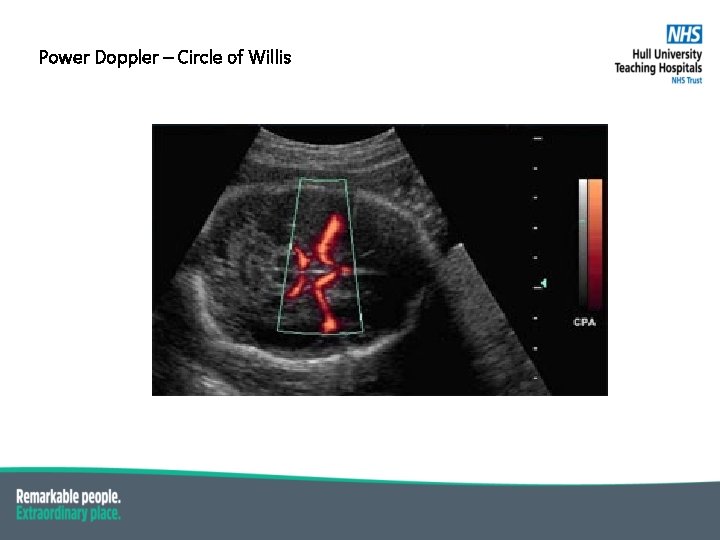
Power Doppler – Circle of Willis Suspicious dark lesion
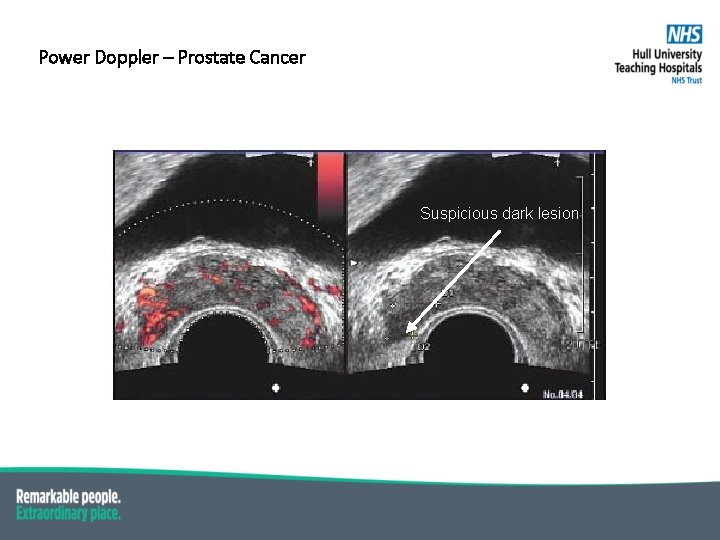
Power Doppler – Prostate Cancer Suspicious dark lesion
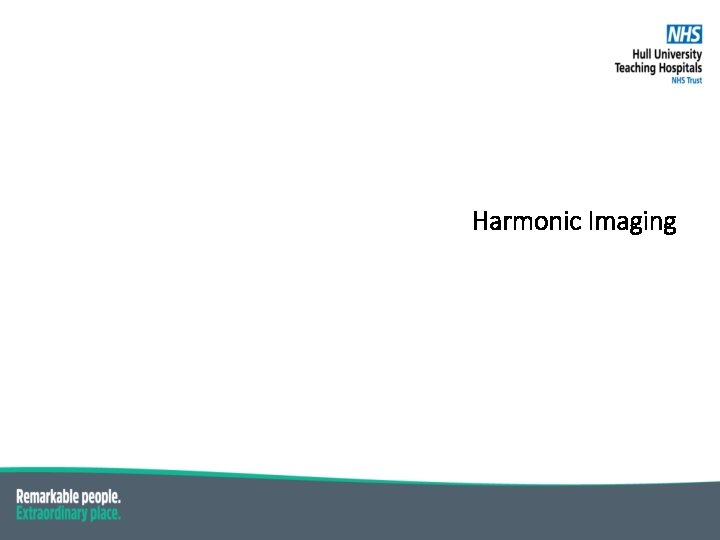
Harmonic Imaging
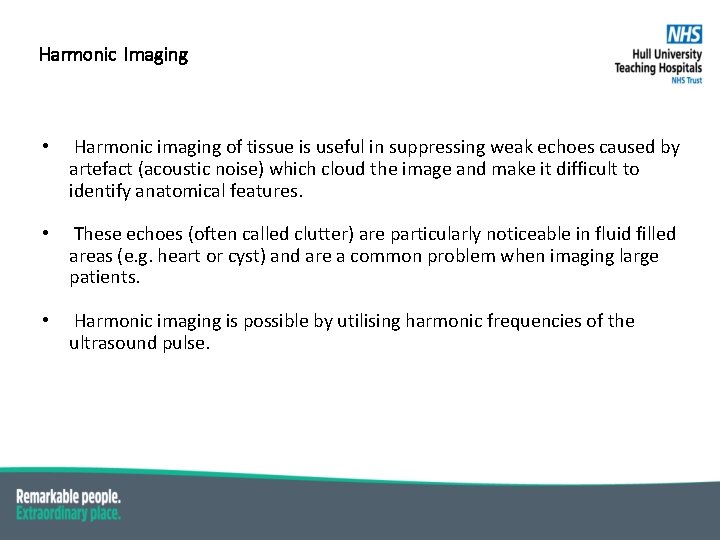
Harmonic Imaging • Harmonic imaging of tissue is useful in suppressing weak echoes caused by artefact (acoustic noise) which cloud the image and make it difficult to identify anatomical features. • These echoes (often called clutter) are particularly noticeable in fluid filled areas (e. g. heart or cyst) and are a common problem when imaging large patients. • Harmonic imaging is possible by utilising harmonic frequencies of the ultrasound pulse.
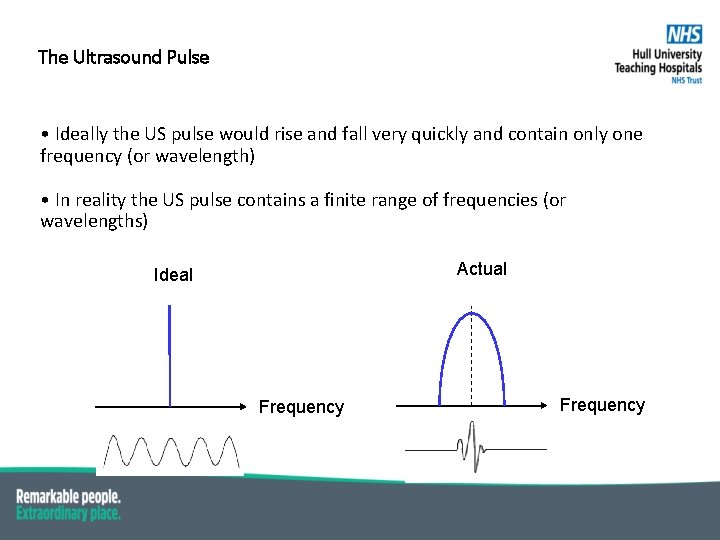
The Ultrasound Pulse • Ideally the US pulse would rise and fall very quickly and contain only one frequency (or wavelength) • In reality the US pulse contains a finite range of frequencies (or wavelengths) Actual Ideal Frequency
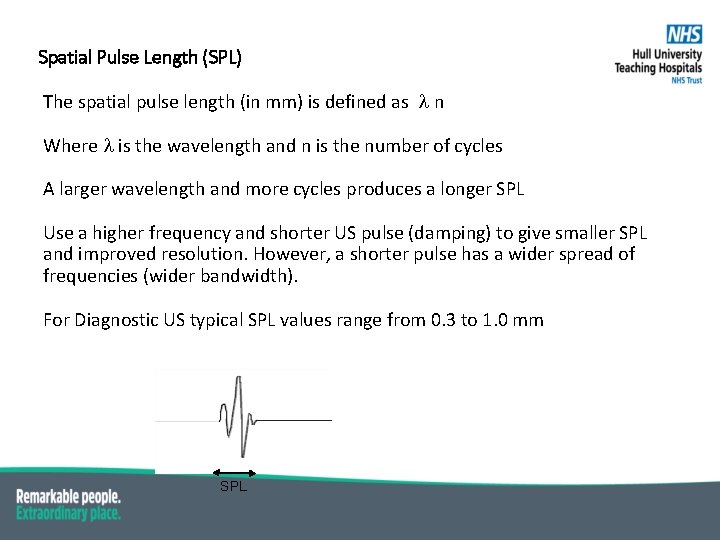
Spatial Pulse Length (SPL) The spatial pulse length (in mm) is defined as n Where is the wavelength and n is the number of cycles A larger wavelength and more cycles produces a longer SPL Use a higher frequency and shorter US pulse (damping) to give smaller SPL and improved resolution. However, a shorter pulse has a wider spread of frequencies (wider bandwidth). For Diagnostic US typical SPL values range from 0. 3 to 1. 0 mm SPL
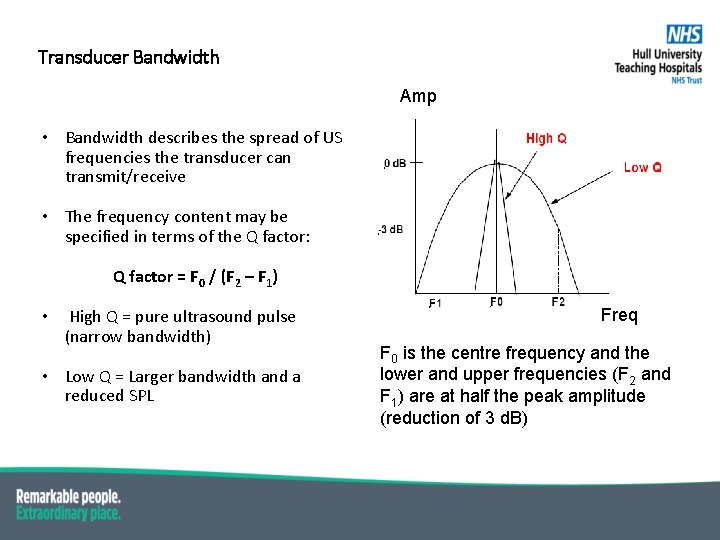
Transducer Bandwidth Amp • Bandwidth describes the spread of US frequencies the transducer can transmit/receive • The frequency content may be specified in terms of the Q factor: Q factor = F 0 / (F 2 – F 1) • High Q = pure ultrasound pulse (narrow bandwidth) • Low Q = Larger bandwidth and a reduced SPL Freq F 0 is the centre frequency and the lower and upper frequencies (F 2 and F 1) are at half the peak amplitude (reduction of 3 d. B)
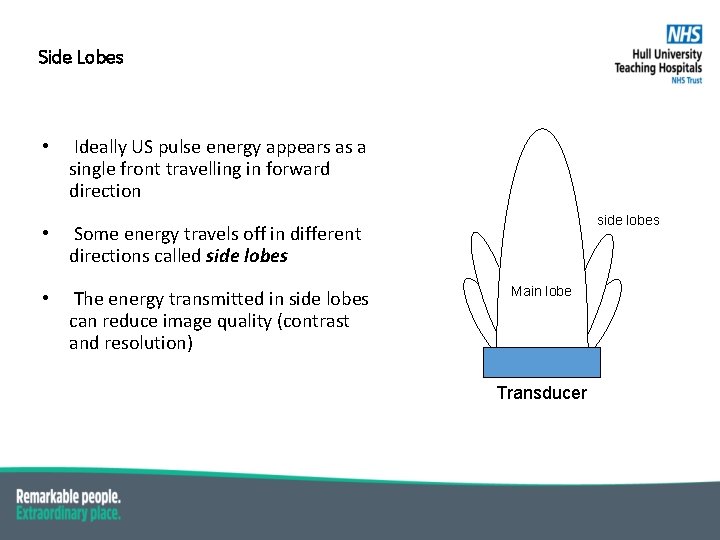
Side Lobes • Ideally US pulse energy appears as a single front travelling in forward direction • Some energy travels off in different directions called side lobes • The energy transmitted in side lobes can reduce image quality (contrast and resolution) side lobes Main lobe Transducer
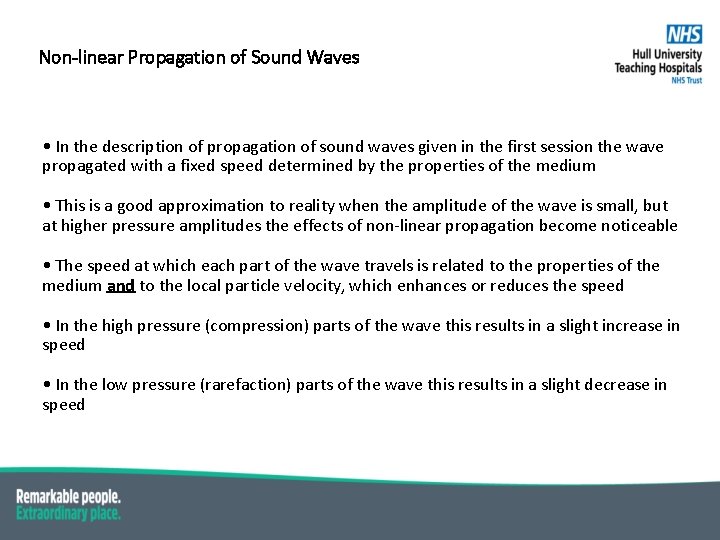
Non-linear Propagation of Sound Waves • In the description of propagation of sound waves given in the first session the wave propagated with a fixed speed determined by the properties of the medium • This is a good approximation to reality when the amplitude of the wave is small, but at higher pressure amplitudes the effects of non-linear propagation become noticeable • The speed at which each part of the wave travels is related to the properties of the medium and to the local particle velocity, which enhances or reduces the speed • In the high pressure (compression) parts of the wave this results in a slight increase in speed • In the low pressure (rarefaction) parts of the wave this results in a slight decrease in speed
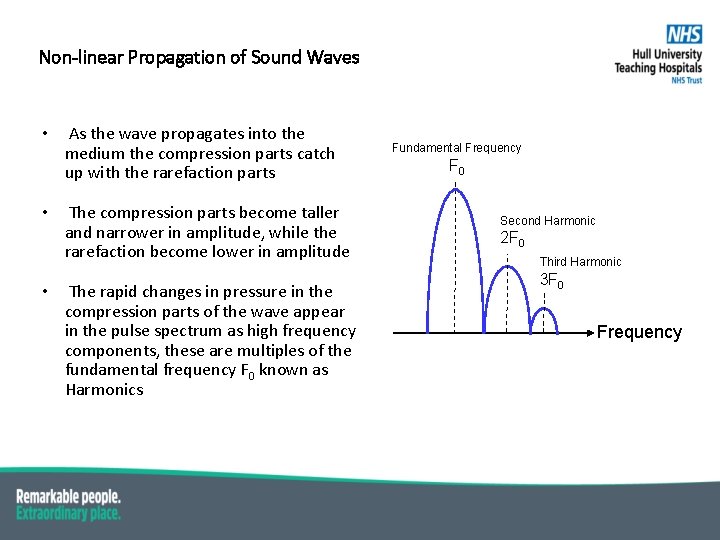
Non-linear Propagation of Sound Waves • • • As the wave propagates into the medium the compression parts catch up with the rarefaction parts The compression parts become taller and narrower in amplitude, while the rarefaction become lower in amplitude The rapid changes in pressure in the compression parts of the wave appear in the pulse spectrum as high frequency components, these are multiples of the fundamental frequency F 0 known as Harmonics Fundamental Frequency F 0 Second Harmonic 2 F 0 Third Harmonic 3 F 0 Frequency
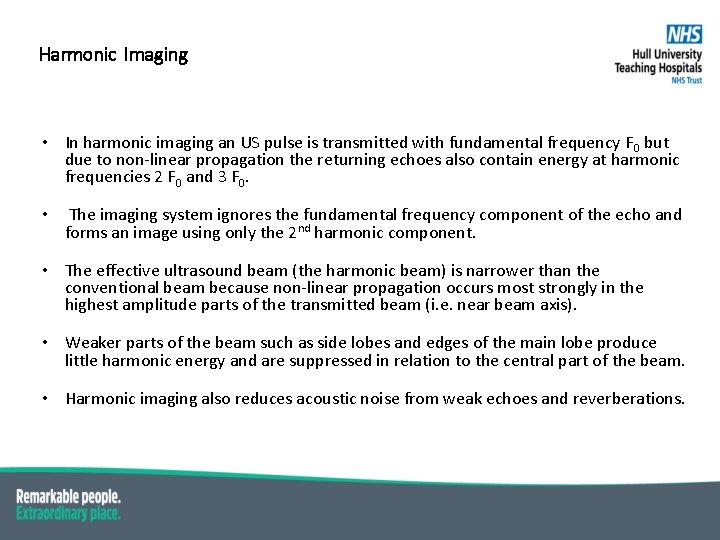
Harmonic Imaging • In harmonic imaging an US pulse is transmitted with fundamental frequency F 0 but due to non-linear propagation the returning echoes also contain energy at harmonic frequencies 2 F 0 and 3 F 0. • The imaging system ignores the fundamental frequency component of the echo and forms an image using only the 2 nd harmonic component. • The effective ultrasound beam (the harmonic beam) is narrower than the conventional beam because non-linear propagation occurs most strongly in the highest amplitude parts of the transmitted beam (i. e. near beam axis). • Weaker parts of the beam such as side lobes and edges of the main lobe produce little harmonic energy and are suppressed in relation to the central part of the beam. • Harmonic imaging also reduces acoustic noise from weak echoes and reverberations.
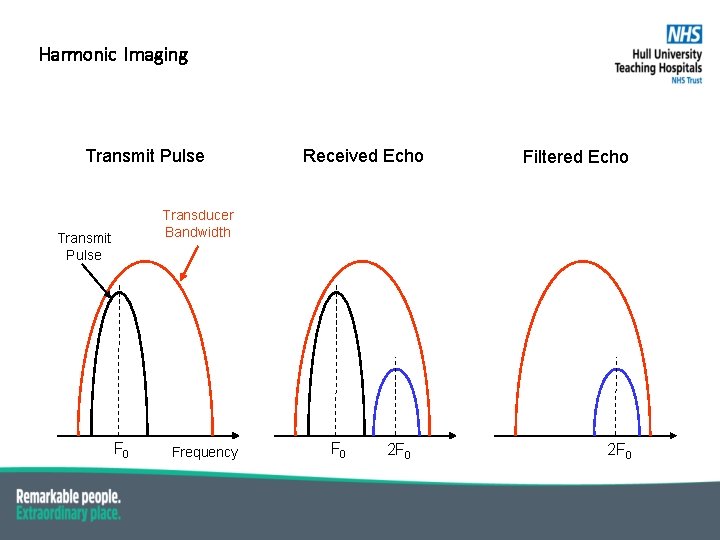
Harmonic Imaging Transmit Pulse Received Echo Filtered Echo Transducer Bandwidth Transmit Pulse F 0 Frequency F 0 2 F 0
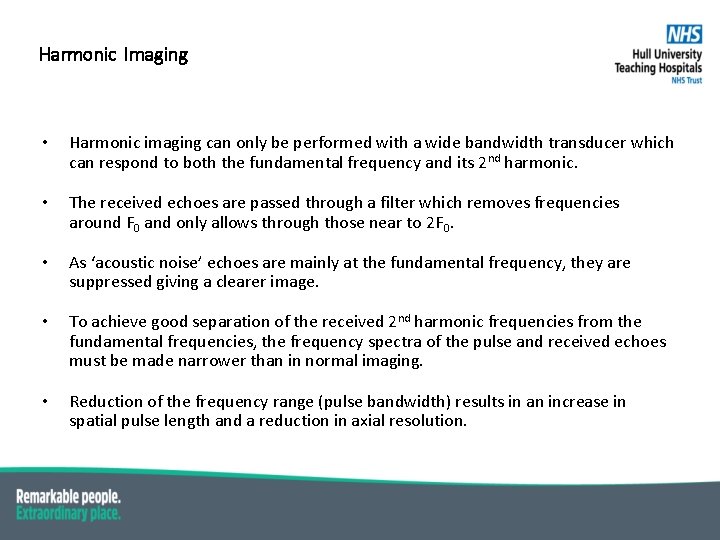
Harmonic Imaging • Harmonic imaging can only be performed with a wide bandwidth transducer which can respond to both the fundamental frequency and its 2 nd harmonic. • The received echoes are passed through a filter which removes frequencies around F 0 and only allows through those near to 2 F 0. • As ‘acoustic noise’ echoes are mainly at the fundamental frequency, they are suppressed giving a clearer image. • To achieve good separation of the received 2 nd harmonic frequencies from the fundamental frequencies, the frequency spectra of the pulse and received echoes must be made narrower than in normal imaging. • Reduction of the frequency range (pulse bandwidth) results in an increase in spatial pulse length and a reduction in axial resolution.
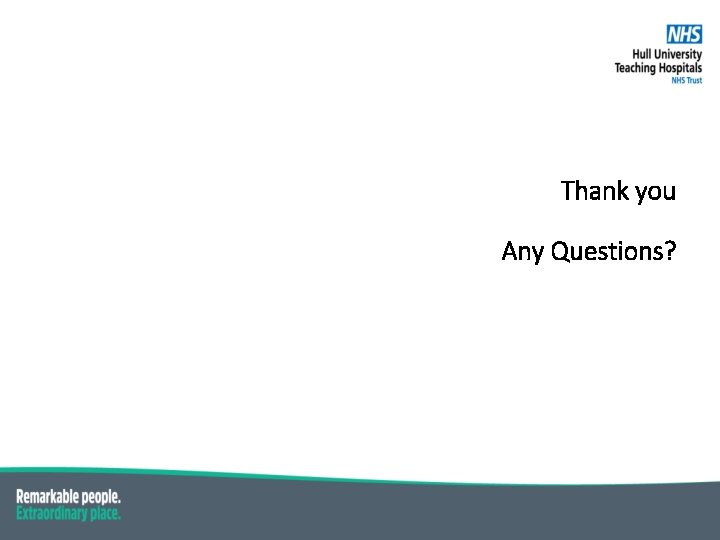
Thank you Any Questions?
Frcr physics lectures
Frequency of xrays
Ferriman-gallwey score
Sound beam ultrasound
Jim kurose lectures
Data mining lectures
Differentiation of rbc
Dr asim lectures
Bba lectures
Cdeep lectures
Activity based approach in software project management
Haematology lectures
Theory of translation lectures
Web engineering lectures ppt
Hugh blair lectures on rhetoric
Bhadeshia lectures
Utilities and energy lecture
Reinforcement learning lectures
What is hegelian philosophy
Medicinal chemistry lectures
Aerodynamics lecture
Ota resident lectures
Medical emergency student lectures
Molecular biology lecture
Bureau of lectures
Theory of translation lectures
Rick trebino lectures
How to get the most out of lectures
Ota resident lectures
Cern summer student lectures
13 lectures
Uva ppt template
Oral communication 3 lectures text
Nuclear medicine lectures
Tamara berg husband
Anatomy lectures powerpoint
Medicine hematology student lectures
Radio astronomy lectures
Trend lectures
Digital logic design lectures
Neonatology lectures
Introduction to recursion
Pathology lectures for medical students
Reinforcement learning lectures
Ludic space
C programming and numerical analysis an introduction
Cs106b lectures
Power system lectures
Cern summer school lectures
Comsats virtual campus lectures
Rcog cpd
Dr sohail lectures
Theory of translation lectures
A mode ultrasound uses
Coupon para adapaleno
Pelvic ultrasound
Ergonomics ultrasound scanning
Anti radial vs radial ultrasound
Carotid doppler velocity chart
Breast ultrasound
Exago ultrasound price
Anne banfield
Hifu
Fast ultrasound
Banana peel sign ultrasound
Piezoelectric effect ultrasound
Beam ultrasound
Antenatal investigations
Rush protocol
Ultrasound time gain compensation
Ultrasound trch salary
Dynamic frequency tuning ultrasound
Causes of decreased fetal movement
Ultrasound beam attenuation
Pinna below the outer canthus of the eye