REACTION KINETICS AND REACTION MECHANISMS Different levels of
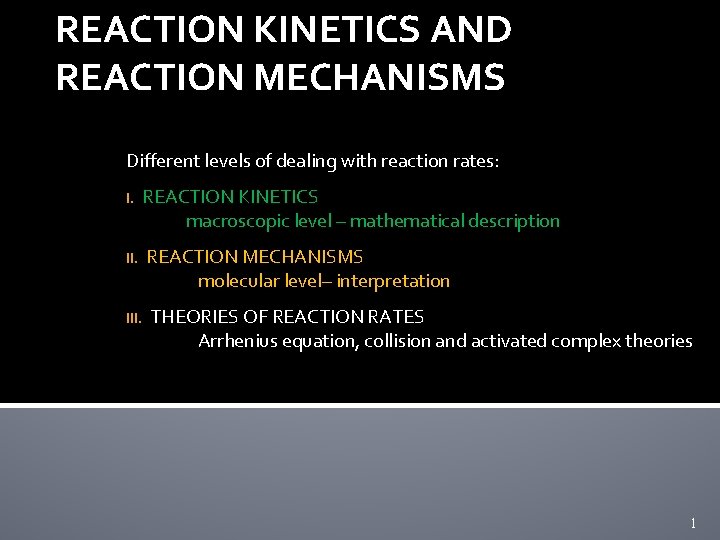
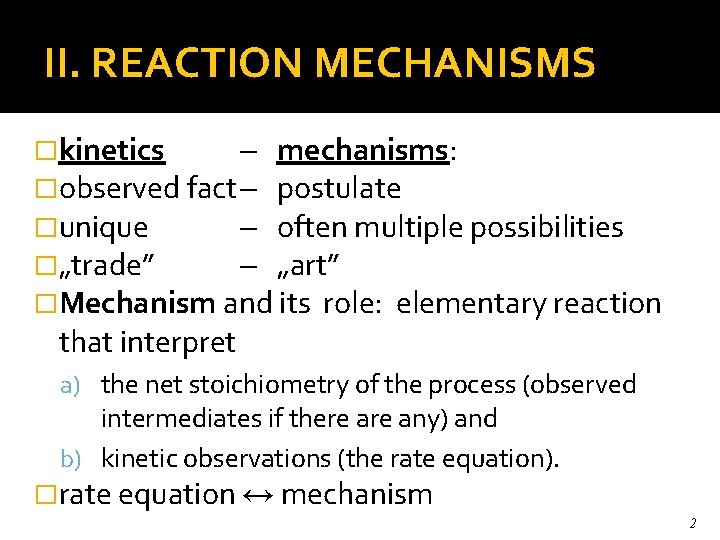
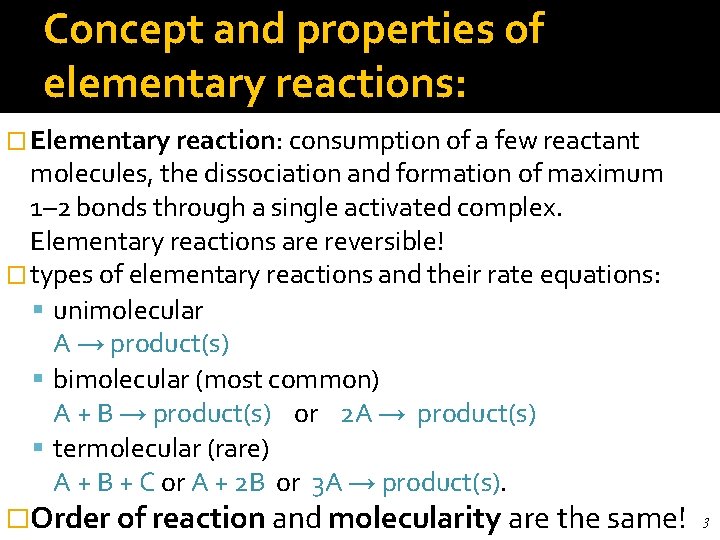
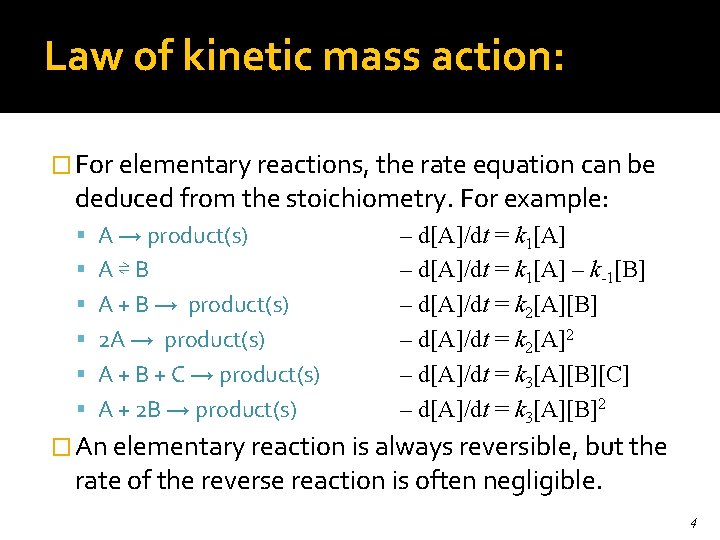
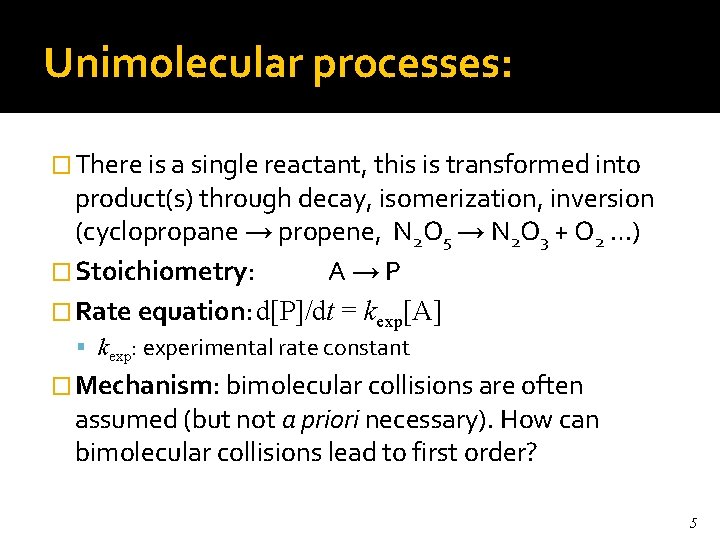
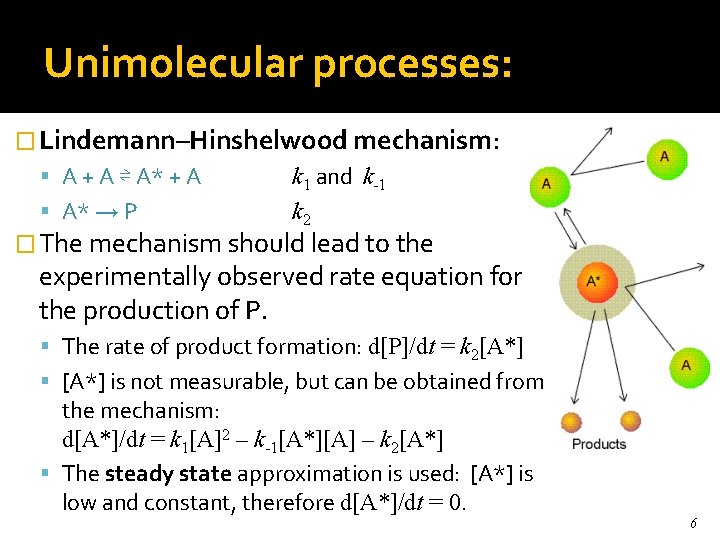
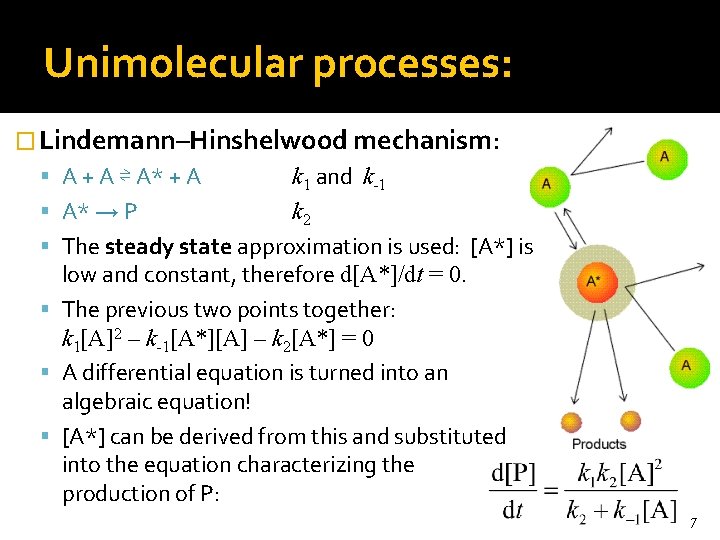
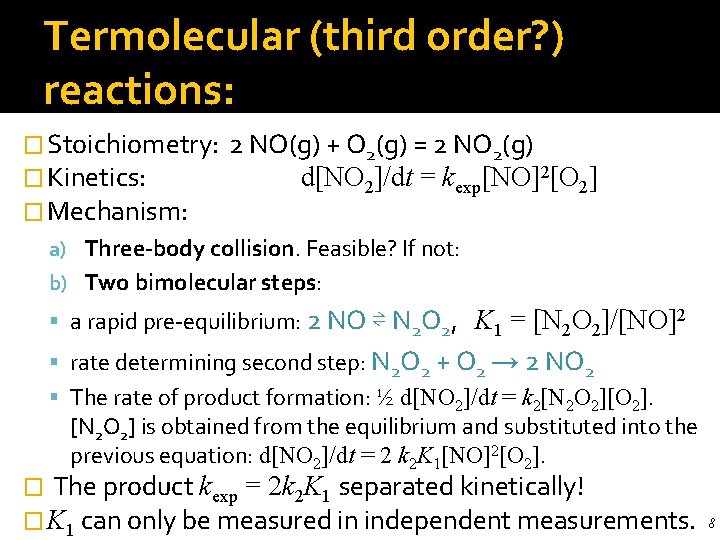
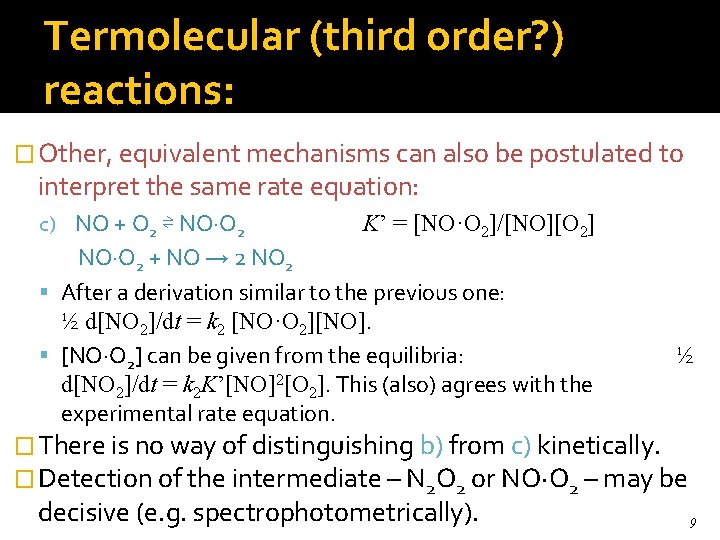
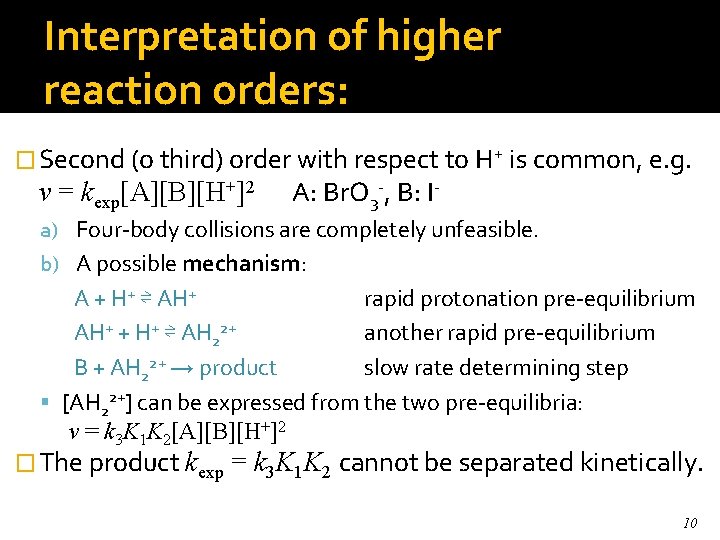
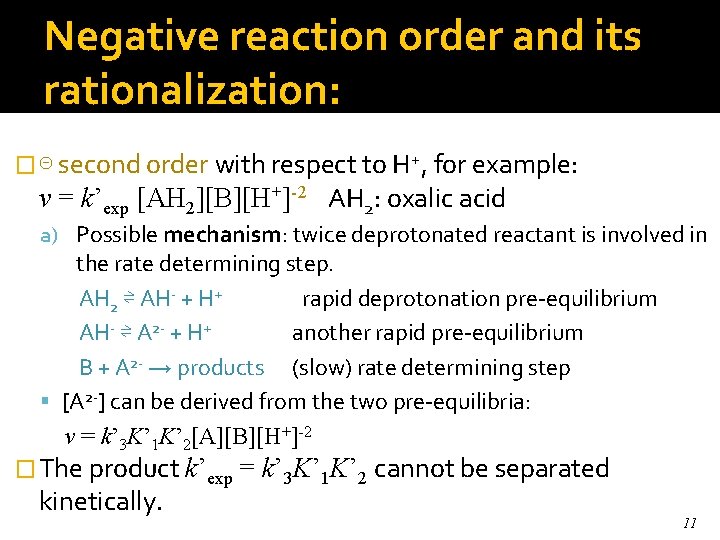
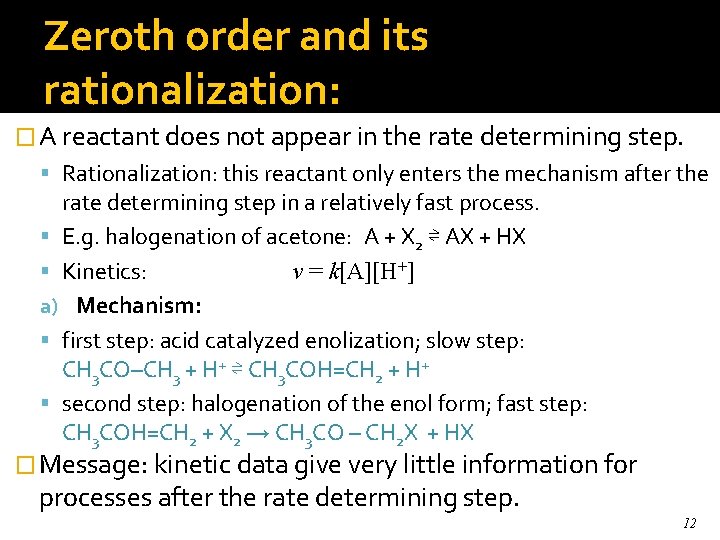
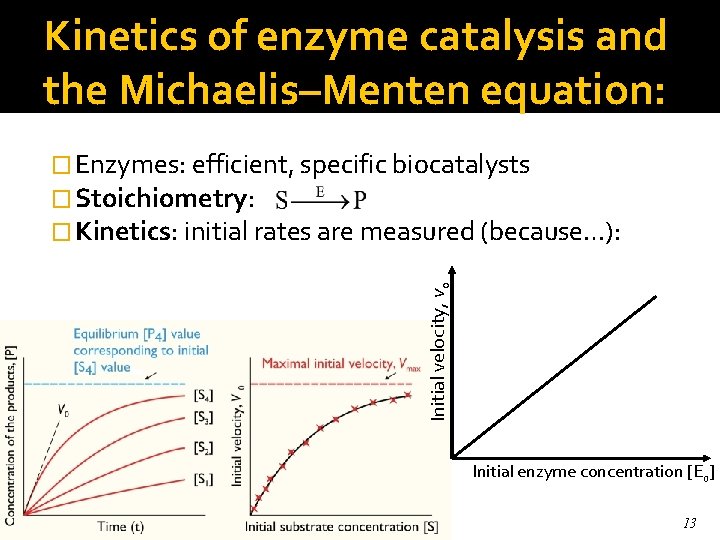
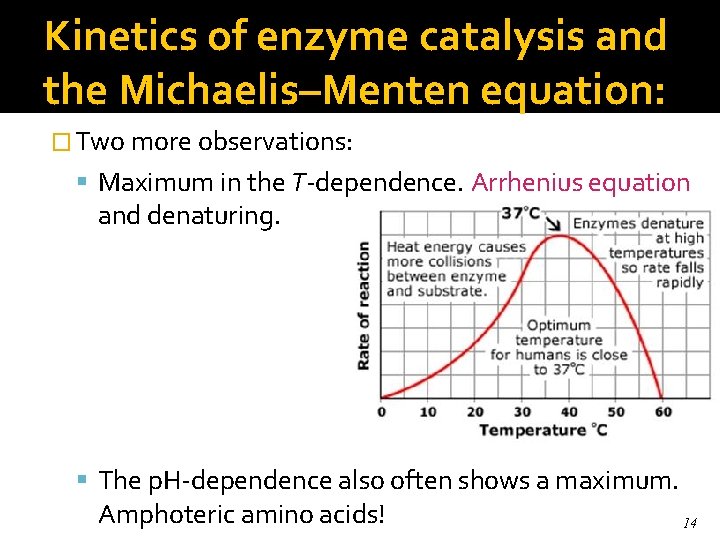
![Kinetics of enzyme catalysis and the Michaelis–Menten equation: � Description of v–[S] curves: or Kinetics of enzyme catalysis and the Michaelis–Menten equation: � Description of v–[S] curves: or](https://slidetodoc.com/presentation_image_h2/8dfc8c0cdd5d03803ee081cea2e304ce/image-15.jpg)
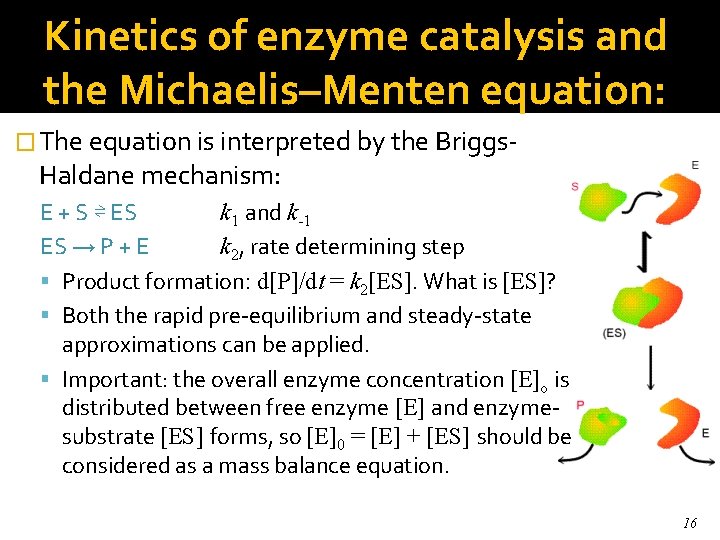
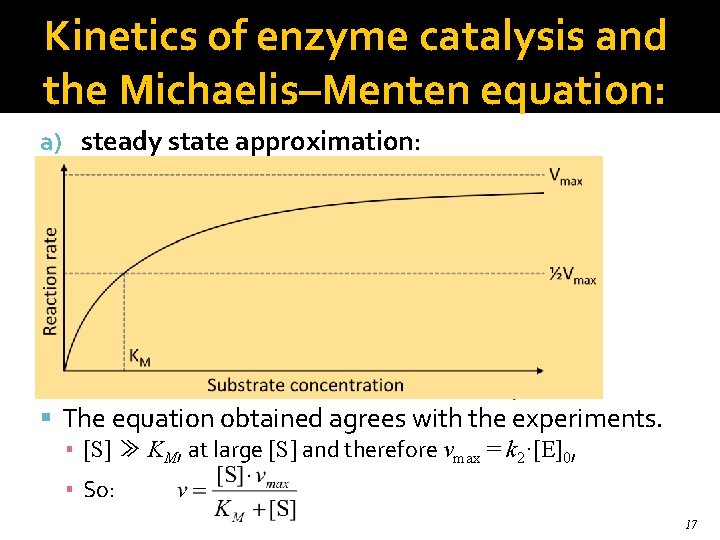
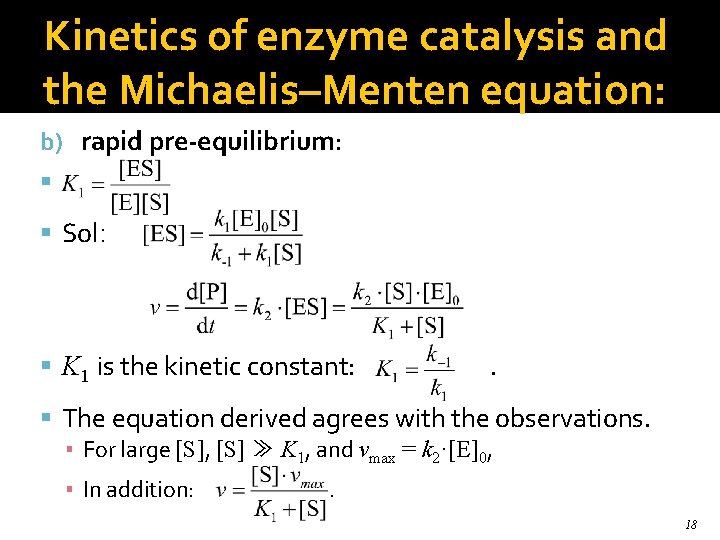
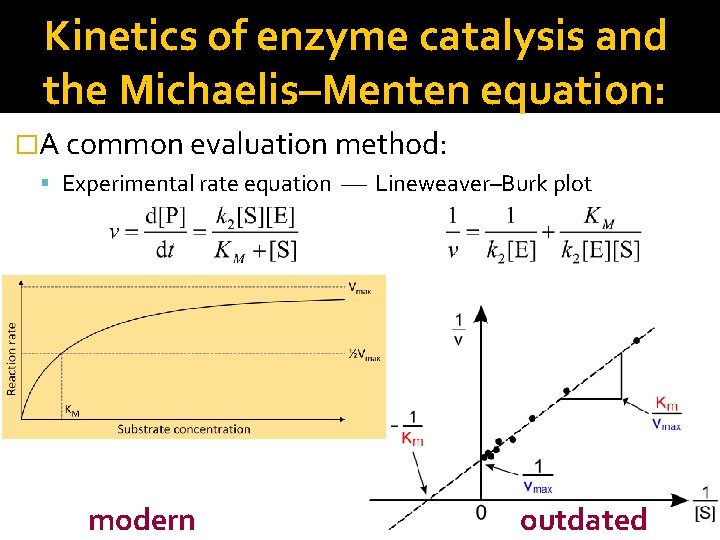
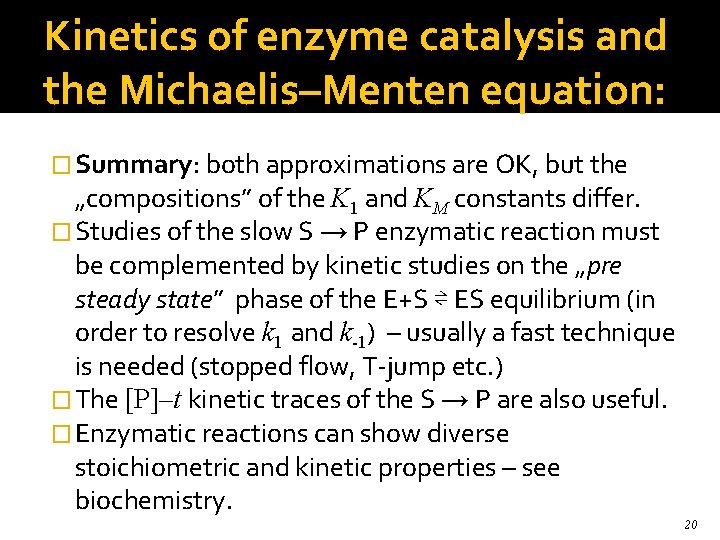
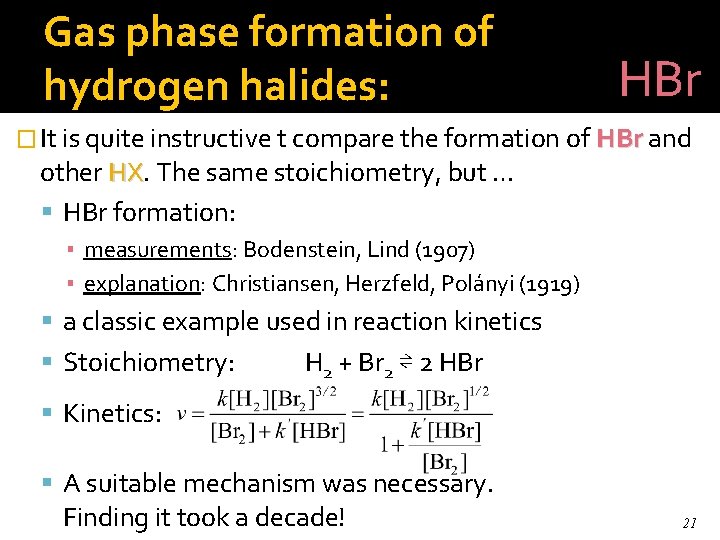
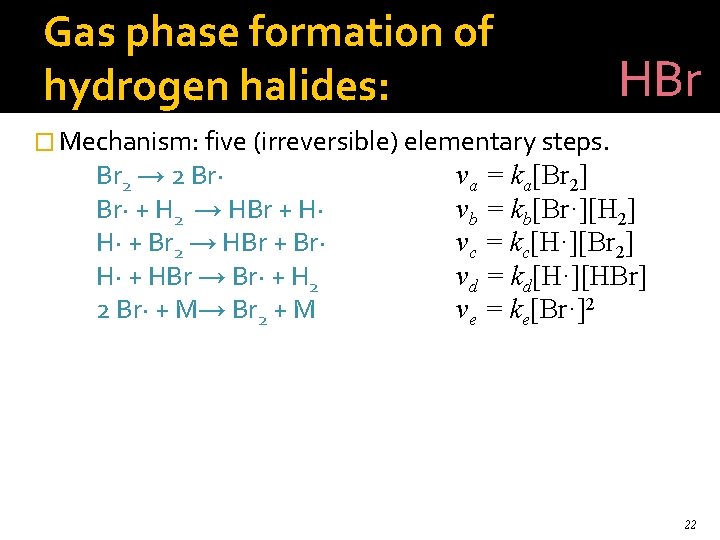
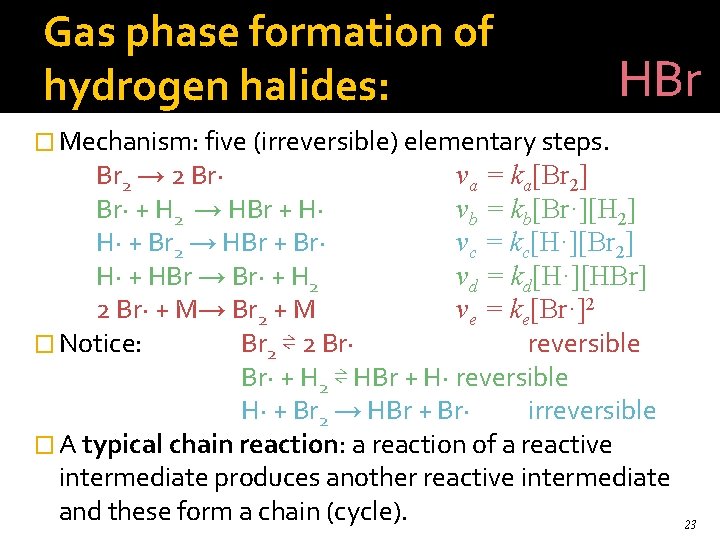
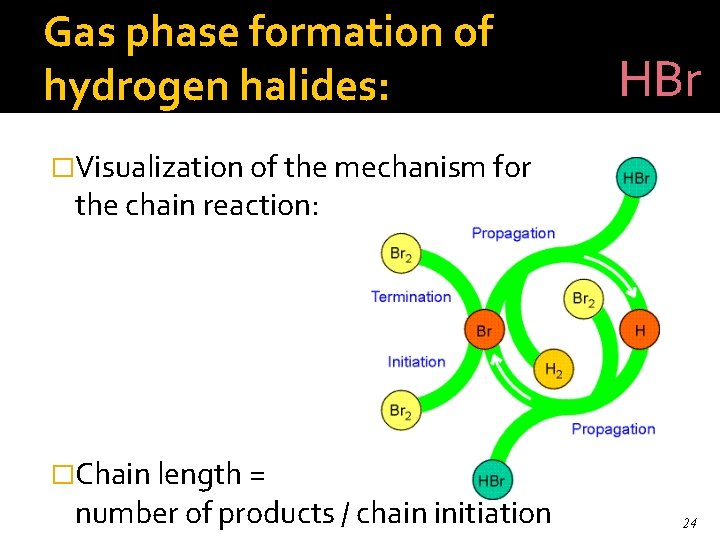
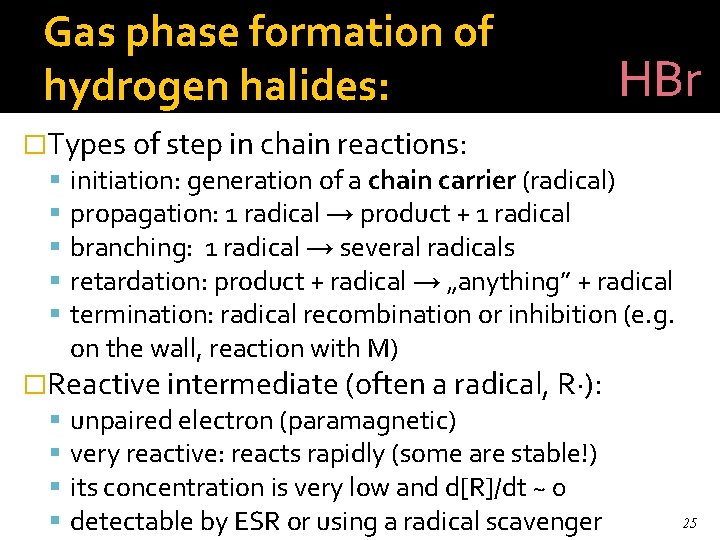
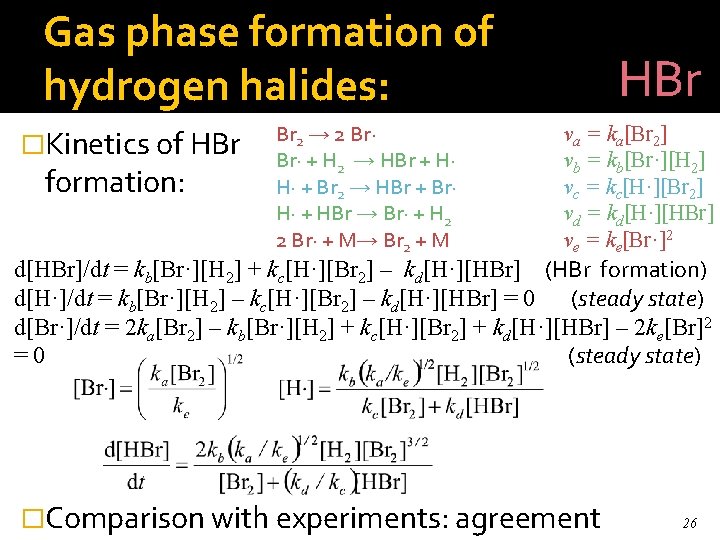
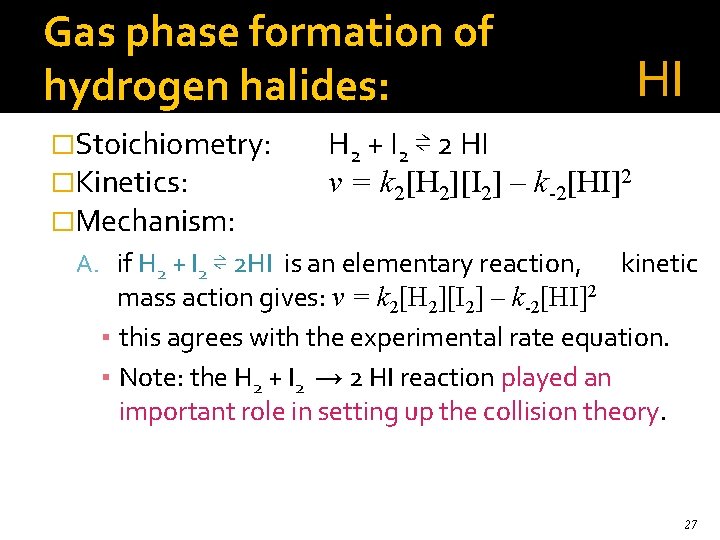
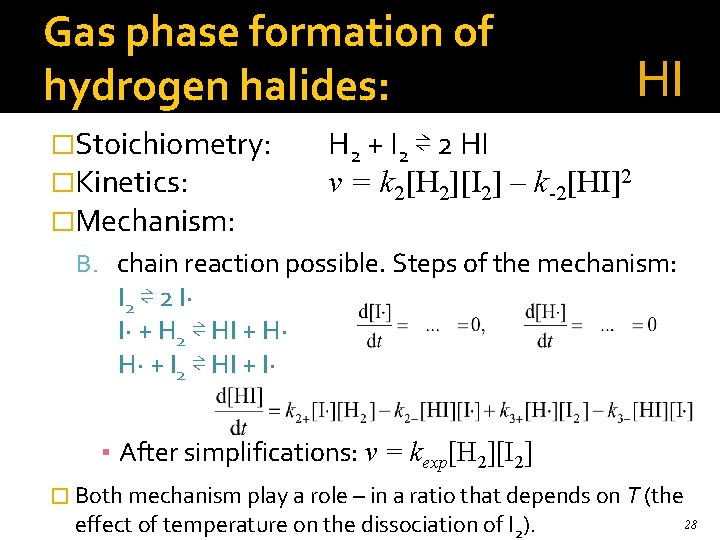
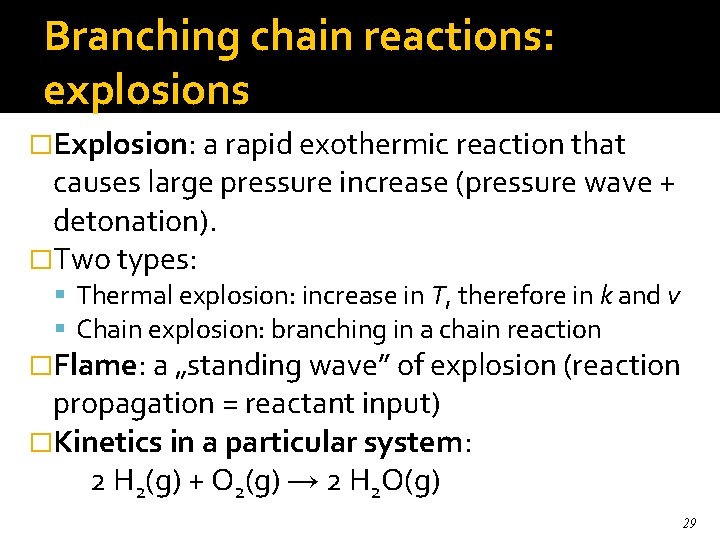
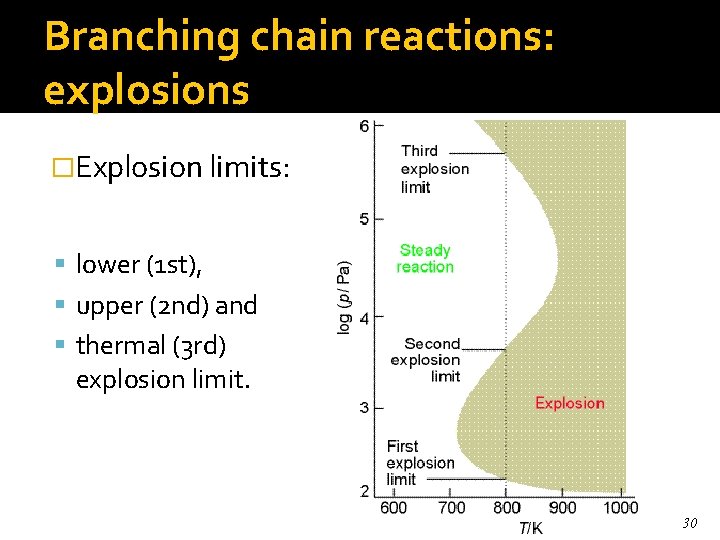
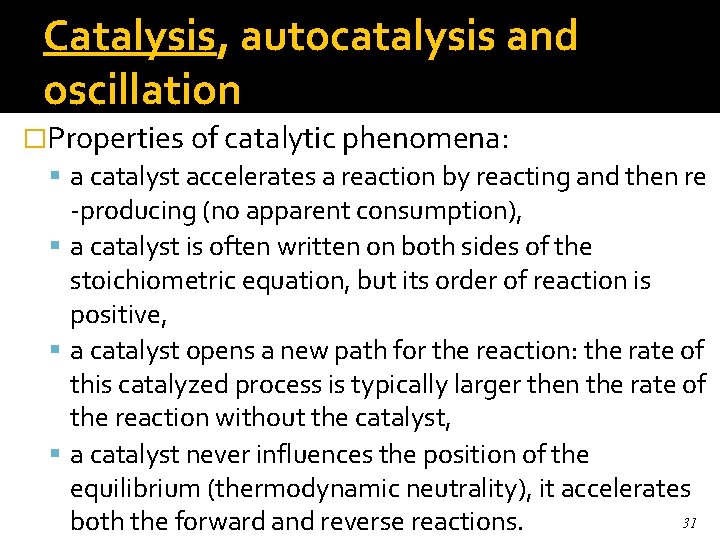
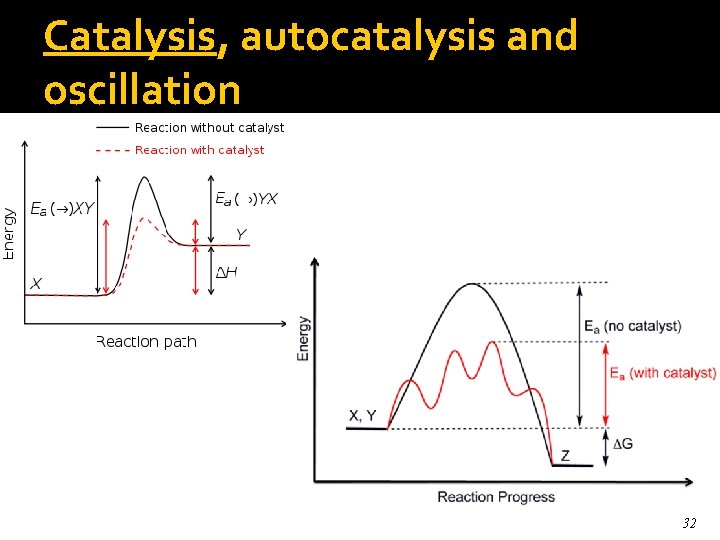
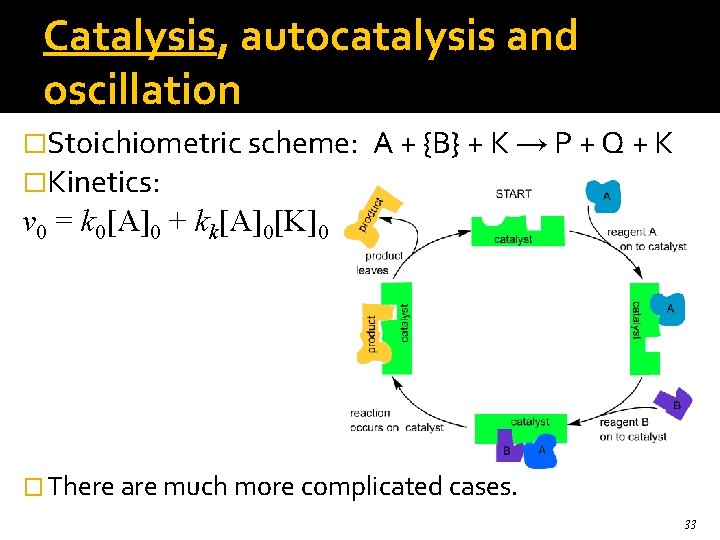
![Catalysis, autocatalysis and oscillation �Autocatalysis: �Stoichiometry: A �Kinetics: v →P = k 0[A] + Catalysis, autocatalysis and oscillation �Autocatalysis: �Stoichiometry: A �Kinetics: v →P = k 0[A] +](https://slidetodoc.com/presentation_image_h2/8dfc8c0cdd5d03803ee081cea2e304ce/image-34.jpg)
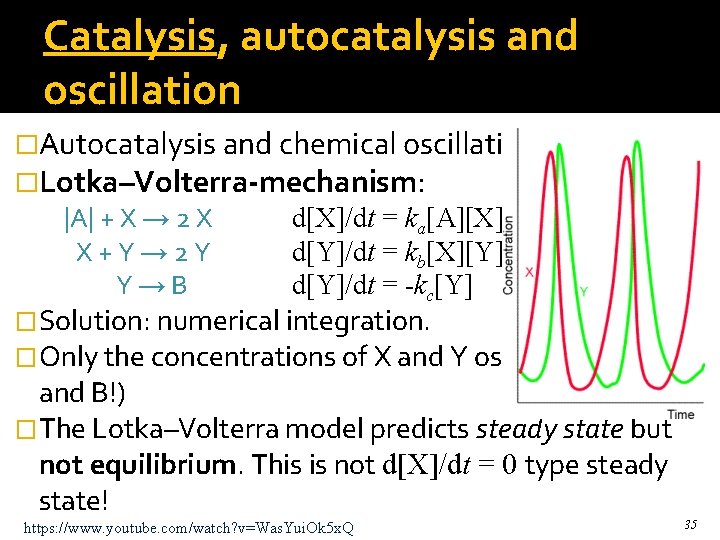
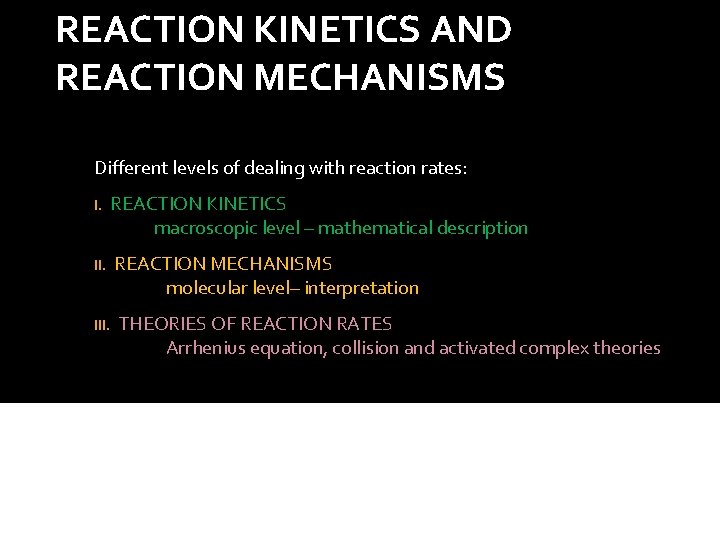
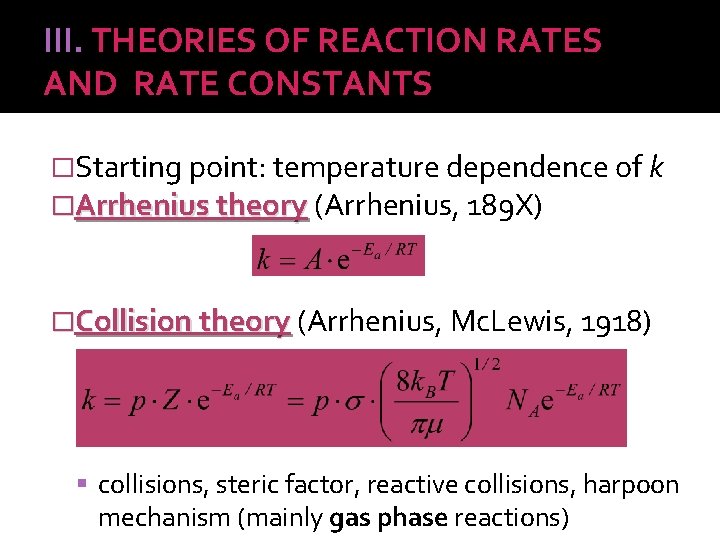
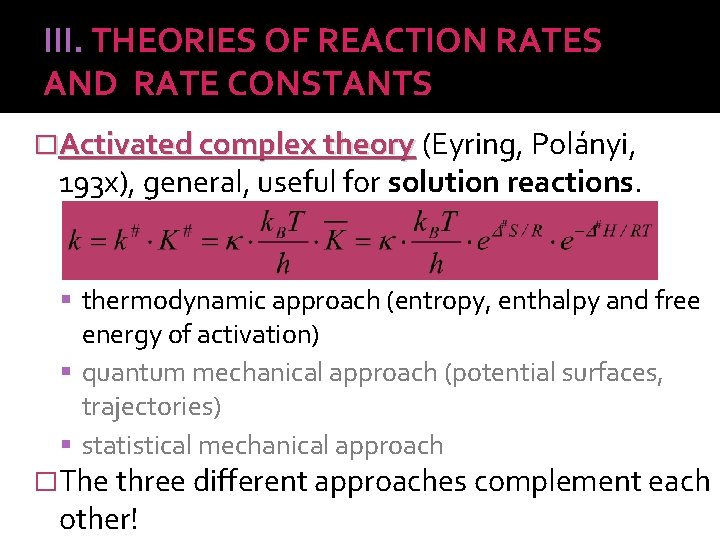
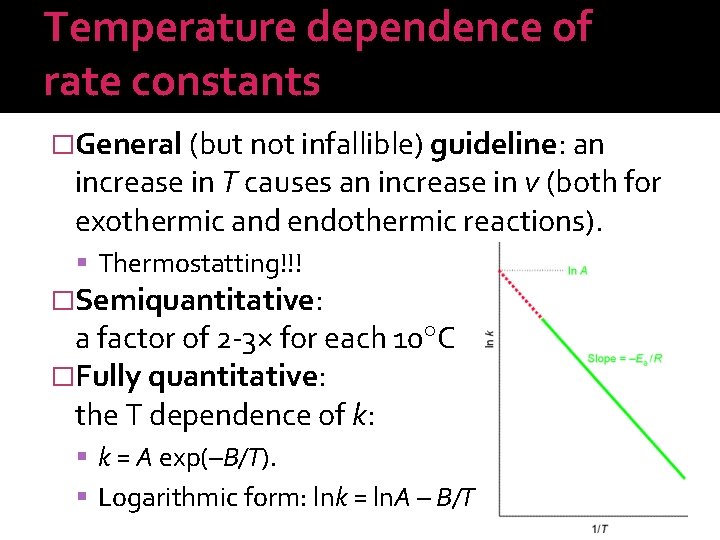
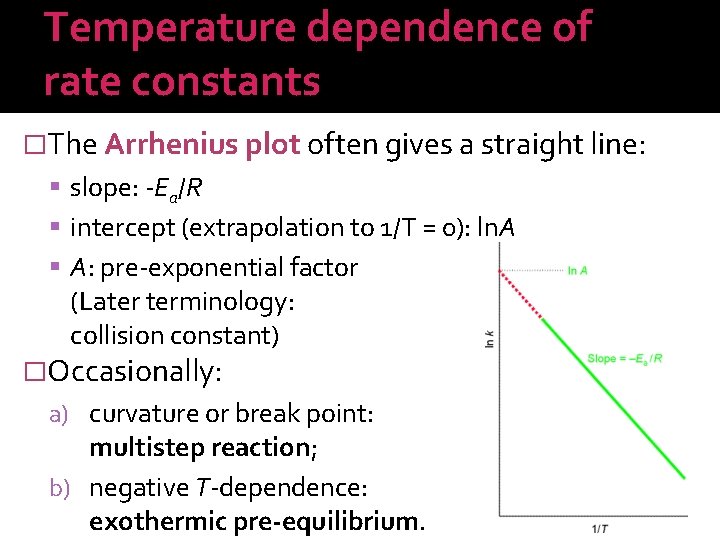
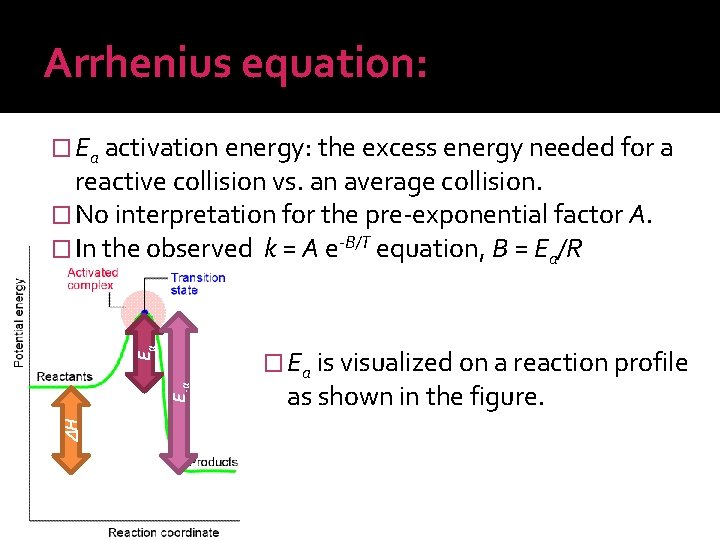
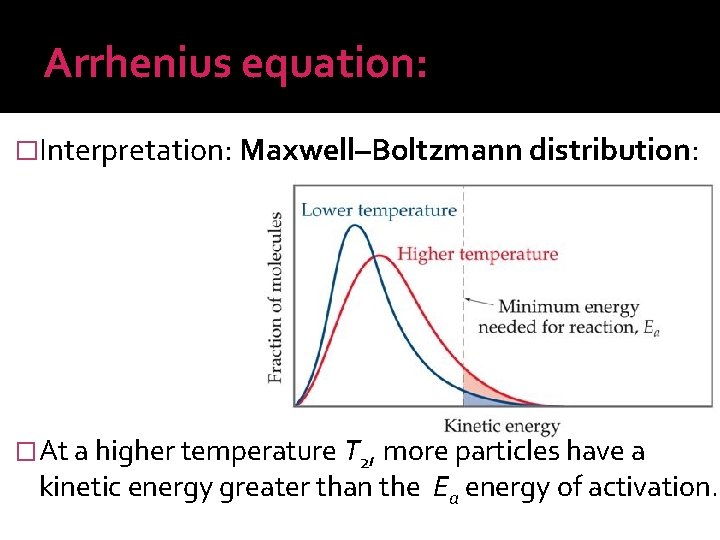
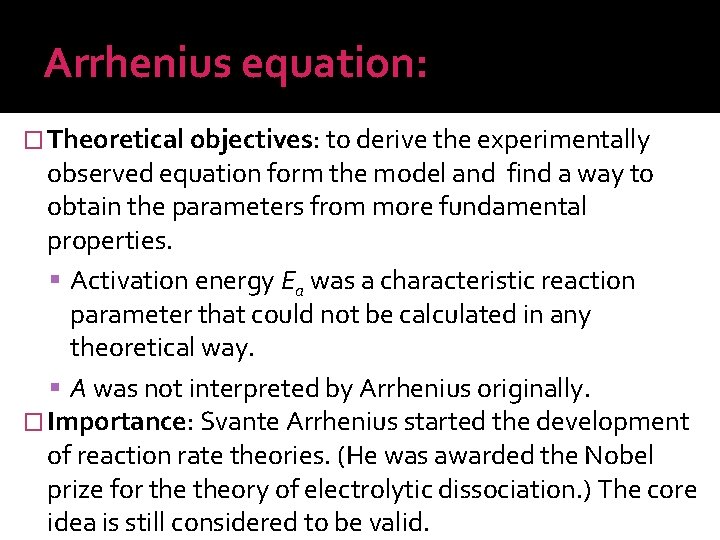
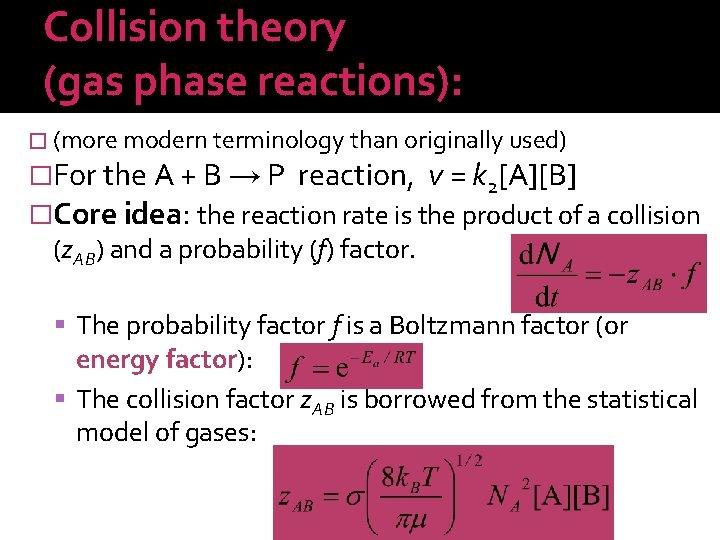
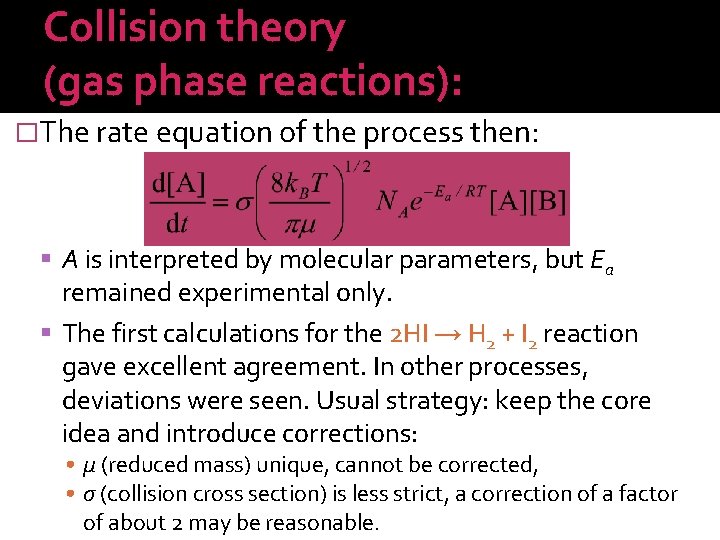
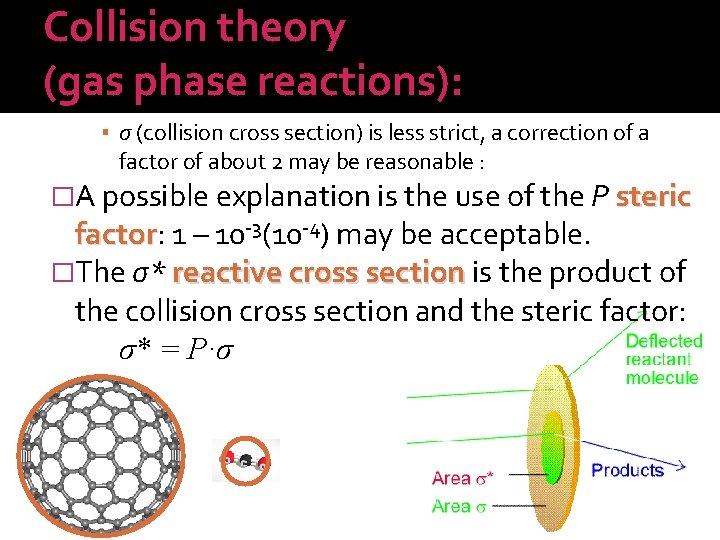
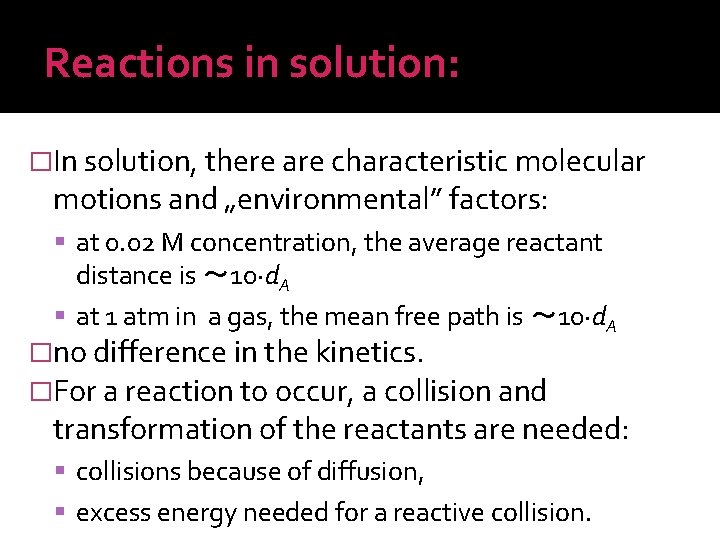
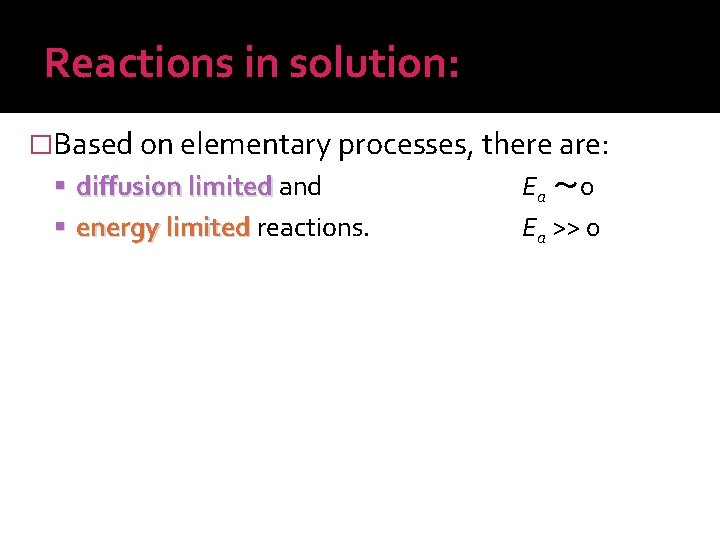
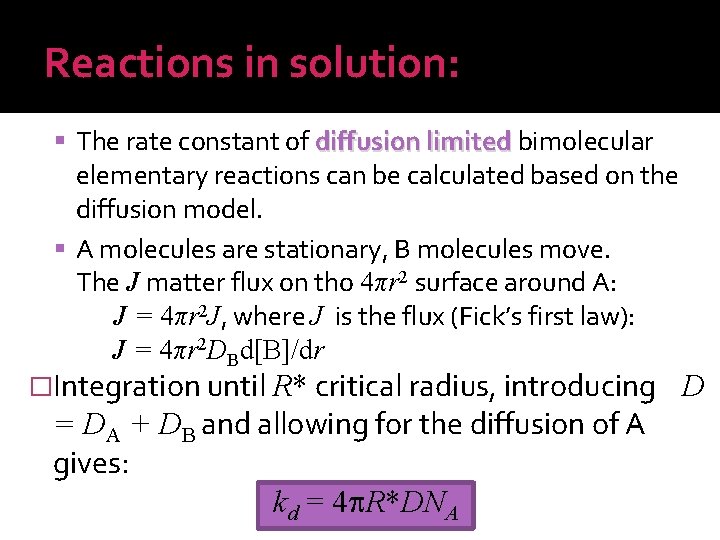
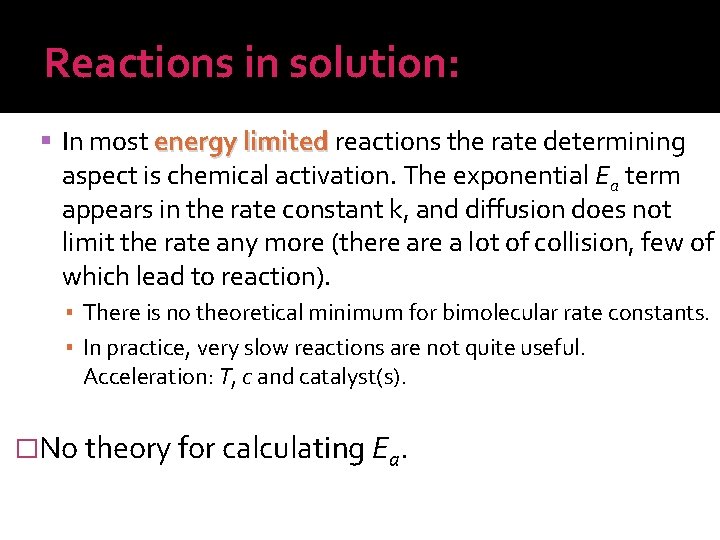
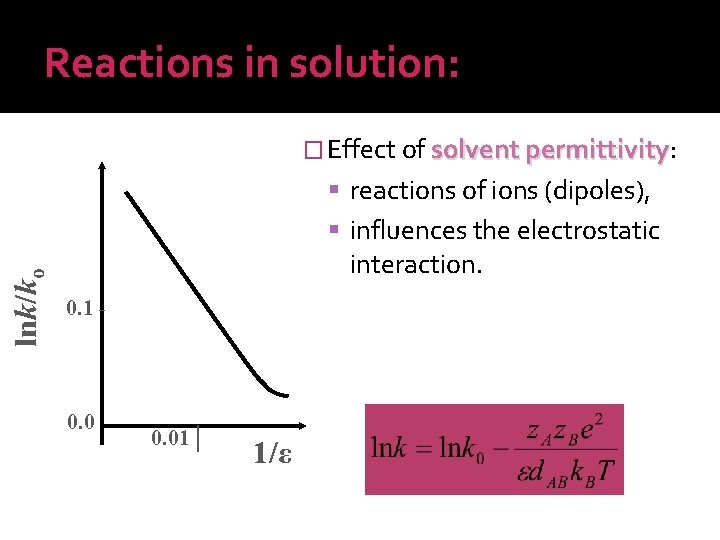
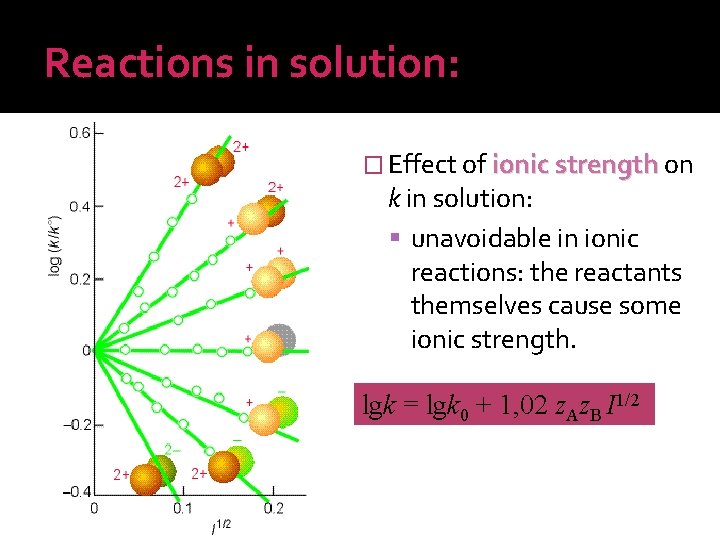
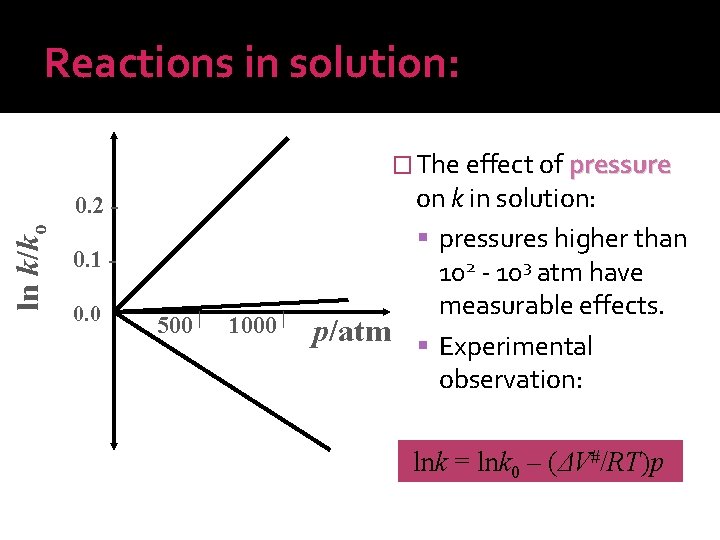
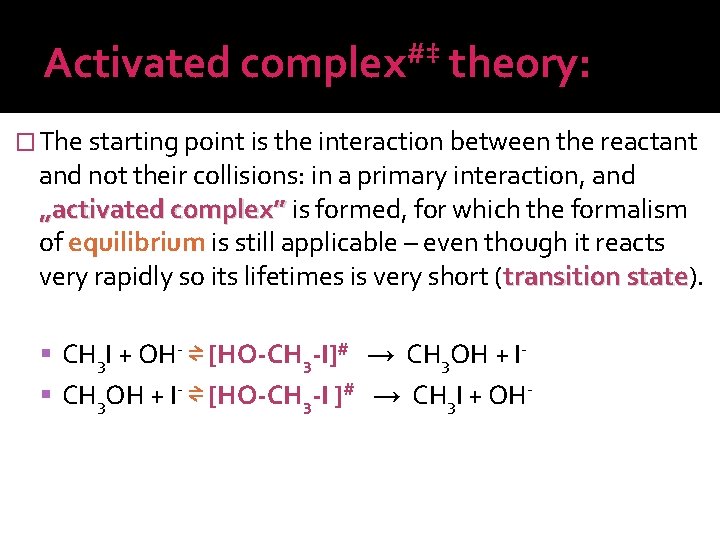
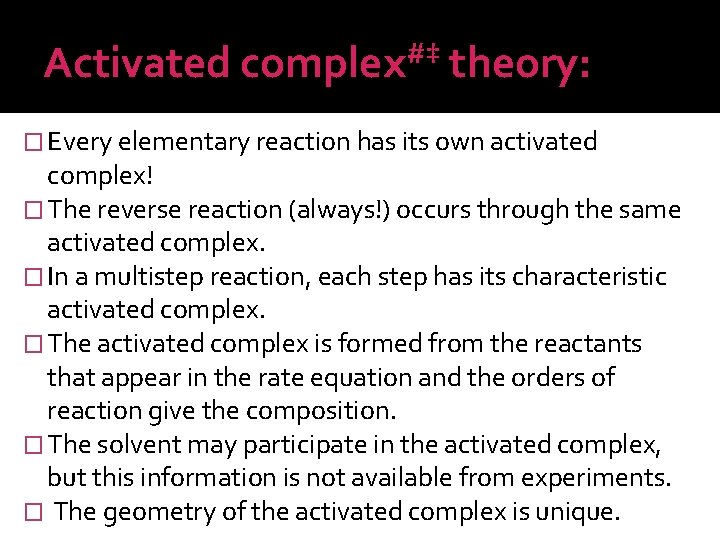
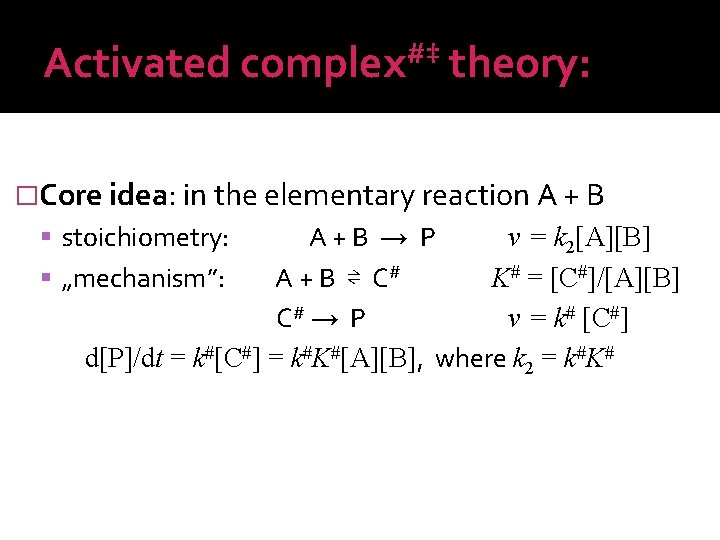
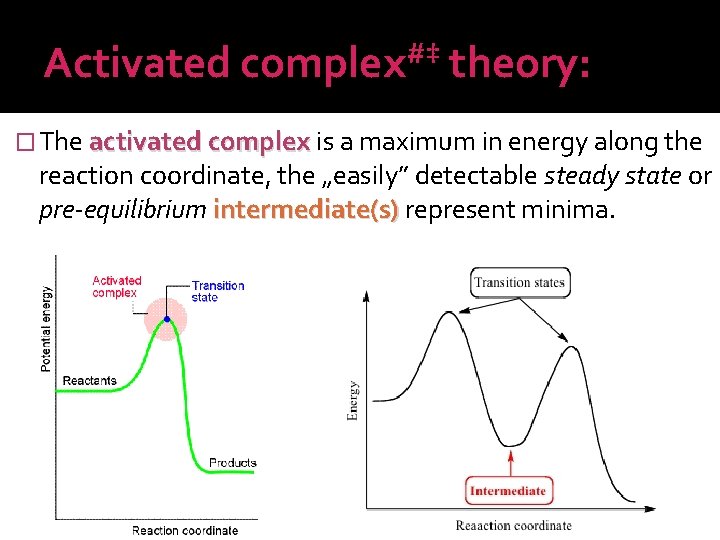
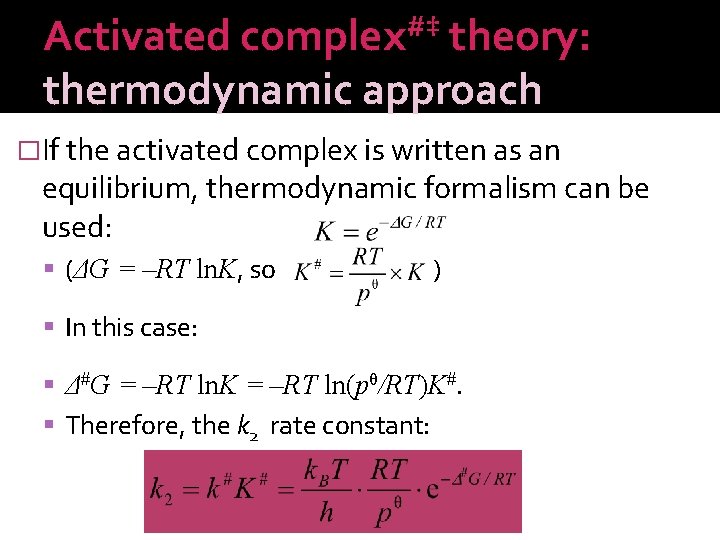
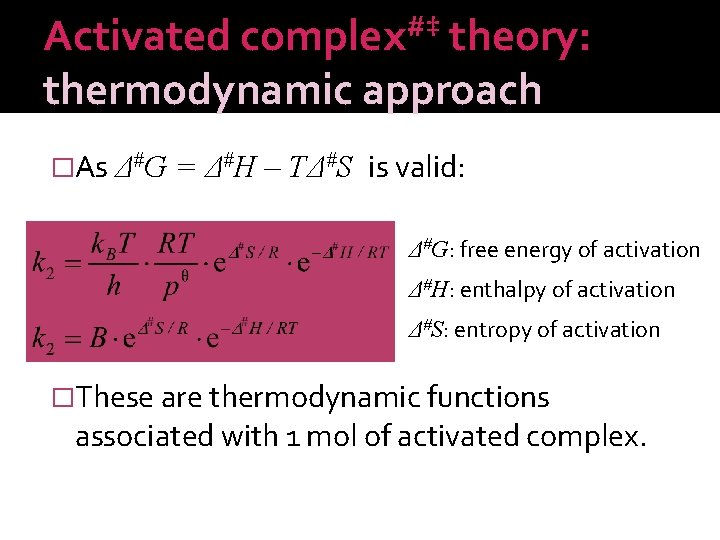
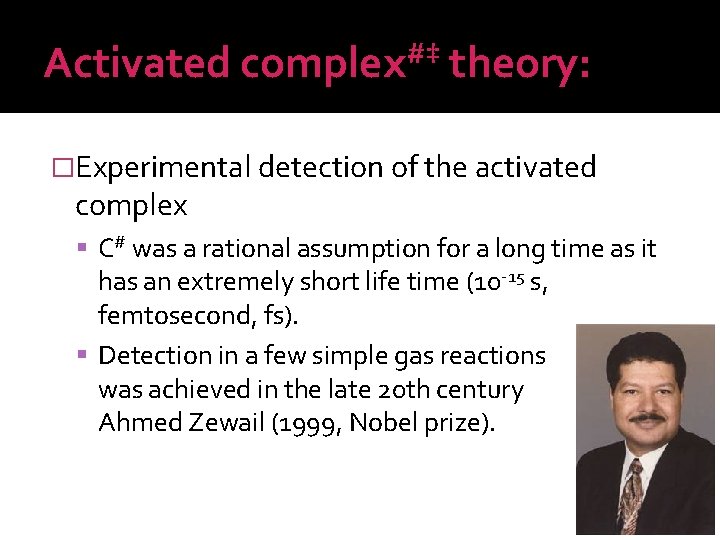
- Slides: 60
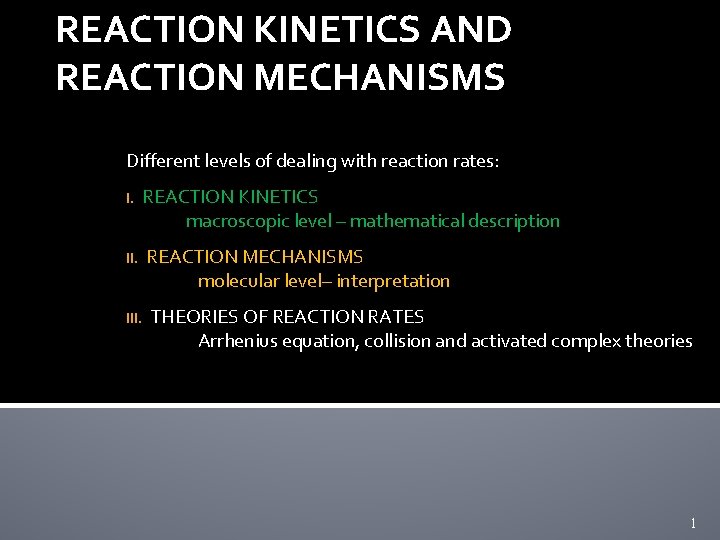
REACTION KINETICS AND REACTION MECHANISMS Different levels of dealing with reaction rates: I. REACTION KINETICS macroscopic level – mathematical description II. REACTION MECHANISMS molecular level– interpretation III. THEORIES OF REACTION RATES Arrhenius equation, collision and activated complex theories 1
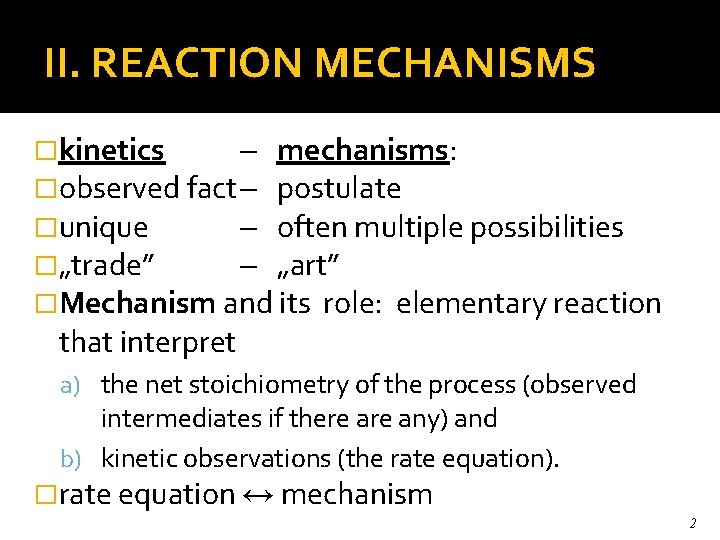
II. REACTION MECHANISMS �kinetics mechanisms: �observed fact postulate �unique often multiple possibilities �„trade” „art” �Mechanism and its role: elementary reaction that interpret a) the net stoichiometry of the process (observed intermediates if there any) and b) kinetic observations (the rate equation). �rate equation ↔ mechanism 2
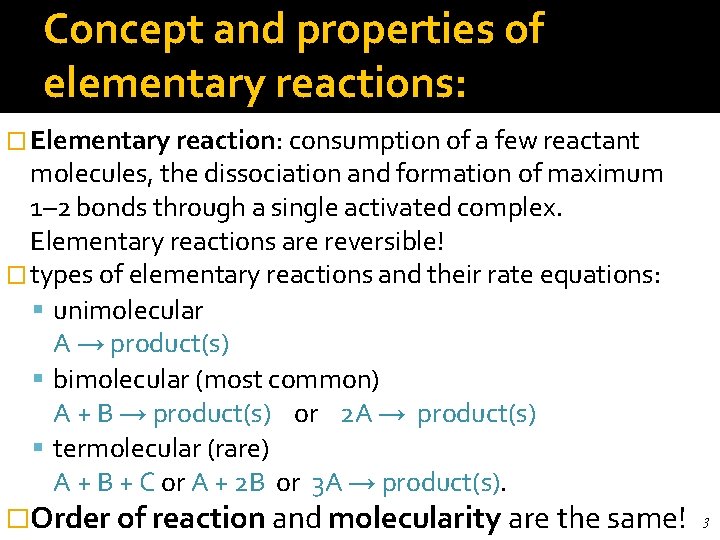
Concept and properties of elementary reactions: � Elementary reaction: consumption of a few reactant molecules, the dissociation and formation of maximum 1– 2 bonds through a single activated complex. Elementary reactions are reversible! � types of elementary reactions and their rate equations: unimolecular A → product(s) bimolecular (most common) A + B → product(s) or 2 A → product(s) termolecular (rare) A + B + C or A + 2 B or 3 A → product(s). �Order of reaction and molecularity are the same! 3
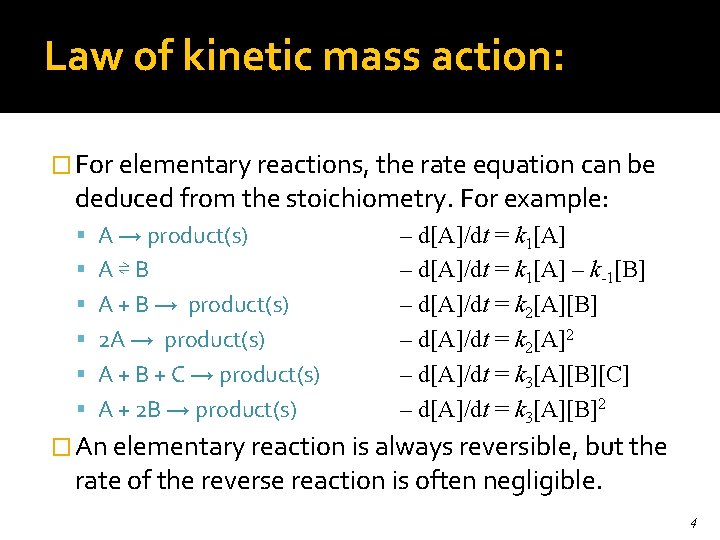
Law of kinetic mass action: � For elementary reactions, the rate equation can be deduced from the stoichiometry. For example: A → product(s) A⇌B A + B → product(s) 2 A → product(s) A + B + C → product(s) A + 2 B → product(s) – d[A]/dt = k 1[A] – k-1[B] – d[A]/dt = k 2[A]2 – d[A]/dt = k 3[A][B][C] – d[A]/dt = k 3[A][B]2 � An elementary reaction is always reversible, but the rate of the reverse reaction is often negligible. 4
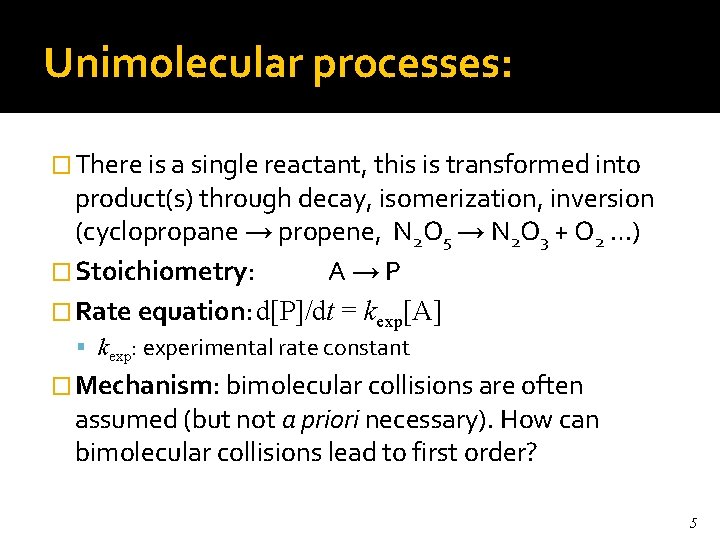
Unimolecular processes: � There is a single reactant, this is transformed into product(s) through decay, isomerization, inversion (cyclopropane → propene, N 2 O 5 → N 2 O 3 + O 2 …) � Stoichiometry: A→P � Rate equation: d[P]/dt = kexp[A] kexp: experimental rate constant � Mechanism: bimolecular collisions are often assumed (but not a priori necessary). How can bimolecular collisions lead to first order? 5
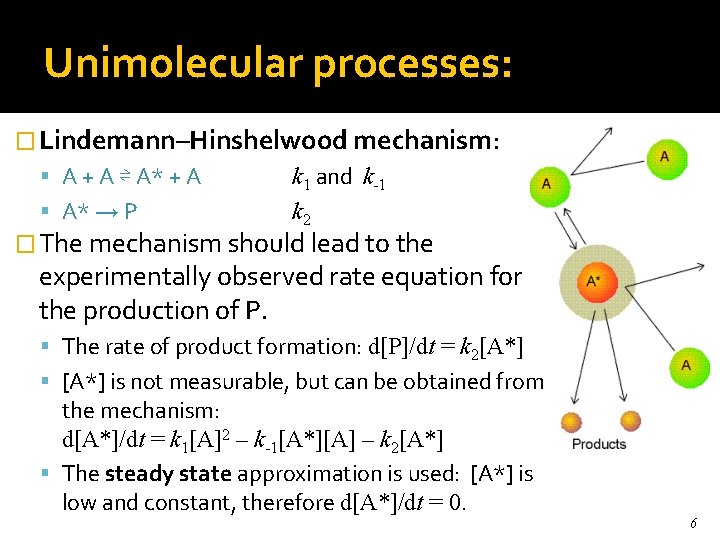
Unimolecular processes: � Lindemann–Hinshelwood mechanism: A + A ⇌ A* + A A* → P k 1 and k-1 k 2 � The mechanism should lead to the experimentally observed rate equation for the production of P. The rate of product formation: d[P]/dt = k 2[A*] is not measurable, but can be obtained from the mechanism: d[A*]/dt = k 1[A]2 – k-1[A*][A] – k 2[A*] The steady state approximation is used: [A*] is low and constant, therefore d[A*]/dt = 0. 6
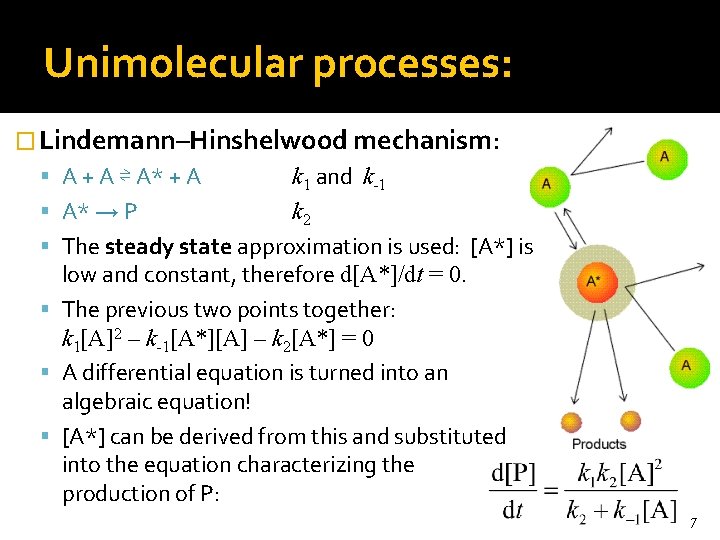
Unimolecular processes: � Lindemann–Hinshelwood mechanism: A + A ⇌ A* + A k 1 and k-1 A* → P k 2 The steady state approximation is used: [A*] is low and constant, therefore d[A*]/dt = 0. The previous two points together: k 1[A]2 – k-1[A*][A] – k 2[A*] = 0 A differential equation is turned into an algebraic equation! [A*] can be derived from this and substituted into the equation characterizing the production of P: 7
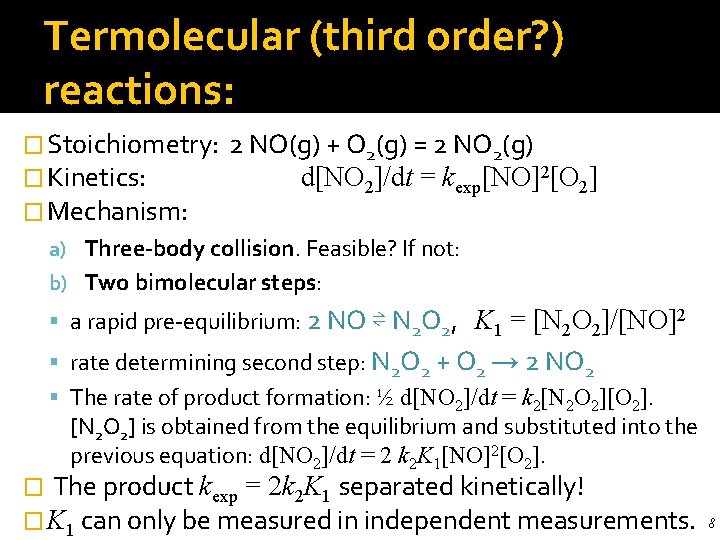
Termolecular (third order? ) reactions: � Stoichiometry: � Kinetics: � Mechanism: 2 NO(g) + O 2(g) = 2 NO 2(g) d[NO 2]/dt = kexp[NO]2[O 2] a) Three-body collision. Feasible? If not: b) Two bimolecular steps: a rapid pre-equilibrium: 2 NO ⇌ N 2 O 2, K 1 = [N 2 O 2]/[NO]2 rate determining second step: N 2 O 2 + O 2 → 2 NO 2 The rate of product formation: ½ d[NO 2]/dt = k 2[N 2 O 2][O 2]. [N 2 O 2] is obtained from the equilibrium and substituted into the previous equation: d[NO 2]/dt = 2 k 2 K 1[NO]2[O 2]. � The product kexp = 2 k 2 K 1 separated kinetically! � K 1 can only be measured in independent measurements. 8
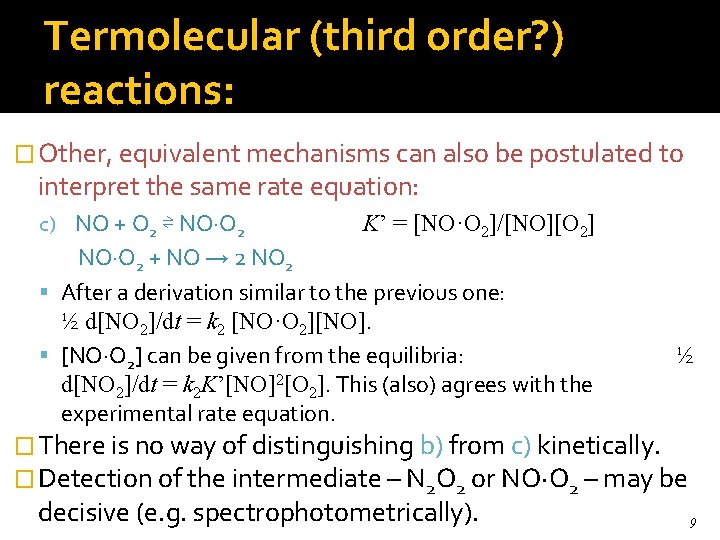
Termolecular (third order? ) reactions: � Other, equivalent mechanisms can also be postulated to interpret the same rate equation: c) NO + O 2 ⇌ NO·O 2 K’ = [NO·O 2]/[NO][O 2] NO·O 2 + NO → 2 NO 2 After a derivation similar to the previous one: ½ d[NO 2]/dt = k 2 [NO·O 2][NO]. [NO·O 2] can be given from the equilibria: d[NO 2]/dt = k 2 K’[NO]2[O 2]. This (also) agrees with the experimental rate equation. ½ � There is no way of distinguishing b) from c) kinetically. � Detection of the intermediate – N 2 O 2 or NO·O 2 – may be decisive (e. g. spectrophotometrically). 9
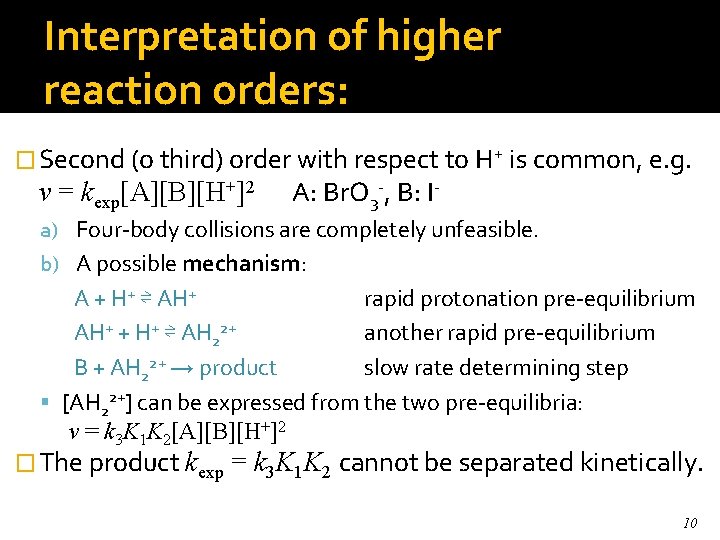
Interpretation of higher reaction orders: � Second (o third) order with respect to H+ is common, e. g. v = kexp[A][B][H+]2 A: Br. O 3 -, B: I- a) Four-body collisions are completely unfeasible. b) A possible mechanism: A + H+ ⇌ AH+ rapid protonation pre-equilibrium AH+ + H+ ⇌ AH 22+ another rapid pre-equilibrium B + AH 22+ → product slow rate determining step [AH 22+] can be expressed from the two pre-equilibria: v = k 3 K 1 K 2[A][B][H+]2 � The product kexp = k 3 K 1 K 2 cannot be separated kinetically. 10
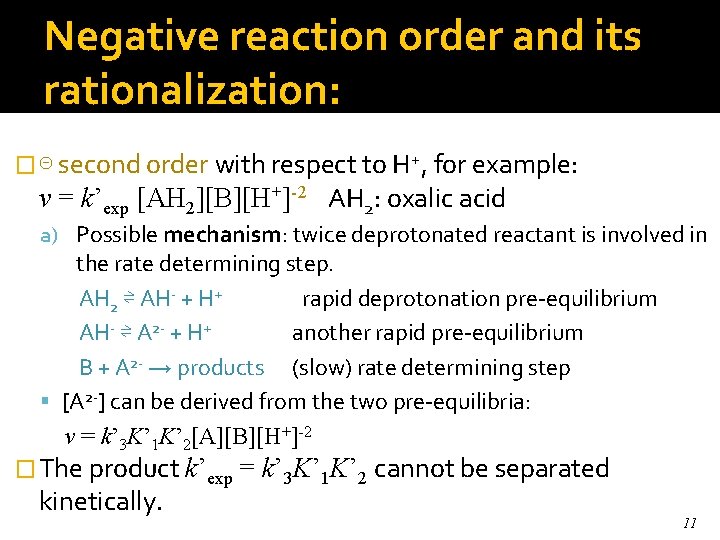
Negative reaction order and its rationalization: � ⊝ second order with respect to H+, for example: v = k’exp [AH 2][B][H+]-2 AH 2: oxalic acid a) Possible mechanism: twice deprotonated reactant is involved in the rate determining step. AH 2 ⇌ AH- + H+ rapid deprotonation pre-equilibrium AH- ⇌ A 2 - + H+ another rapid pre-equilibrium B + A 2 - → products (slow) rate determining step [A 2 -] can be derived from the two pre-equilibria: v = k’ 3 K’ 1 K’ 2[A][B][H+]-2 � The product k’exp = kinetically. k’ 3 K’ 1 K’ 2 cannot be separated 11
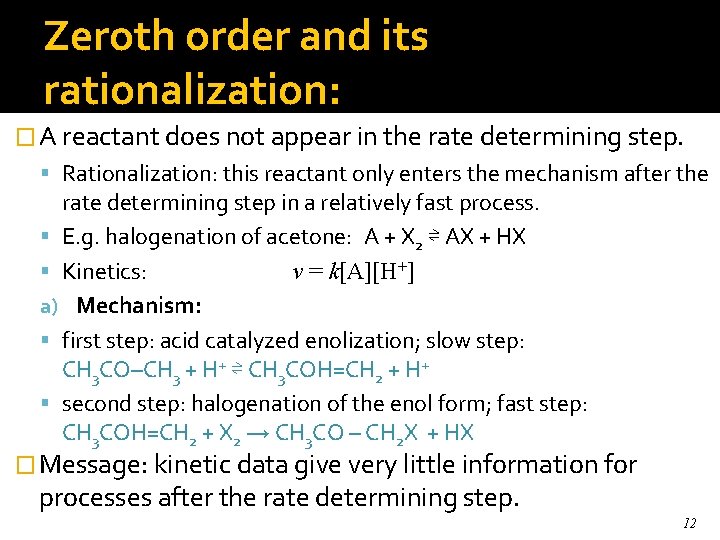
Zeroth order and its rationalization: � A reactant does not appear in the rate determining step. Rationalization: this reactant only enters the mechanism after the rate determining step in a relatively fast process. E. g. halogenation of acetone: A + X 2 ⇌ AX + HX Kinetics: v = k[A][H+] a) Mechanism: first step: acid catalyzed enolization; slow step: CH 3 CO–CH 3 + H+ ⇌ CH 3 COH=CH 2 + H+ second step: halogenation of the enol form; fast step: CH 3 COH=CH 2 + X 2 → CH 3 CO – CH 2 X + HX � Message: kinetic data give very little information for processes after the rate determining step. 12
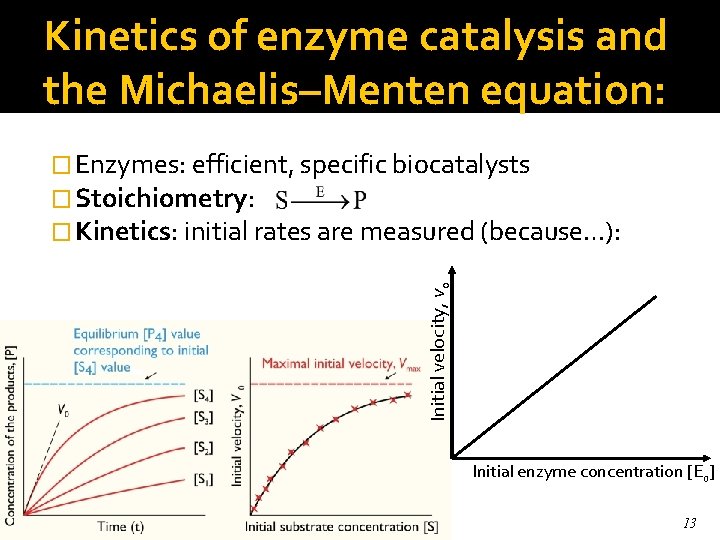
Kinetics of enzyme catalysis and the Michaelis–Menten equation: Initial velocity, vo � Enzymes: efficient, specific biocatalysts � Stoichiometry: � Kinetics: initial rates are measured (because…): Initial enzyme concentration [Eo] 13
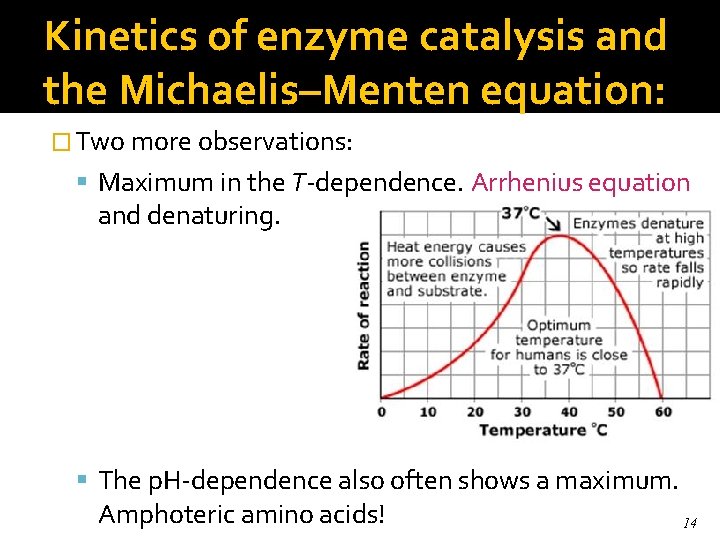
Kinetics of enzyme catalysis and the Michaelis–Menten equation: � Two more observations: Maximum in the T-dependence. Arrhenius equation and denaturing. The p. H-dependence also often shows a maximum. Amphoteric amino acids! 14
![Kinetics of enzyme catalysis and the MichaelisMenten equation Description of vS curves or Kinetics of enzyme catalysis and the Michaelis–Menten equation: � Description of v–[S] curves: or](https://slidetodoc.com/presentation_image_h2/8dfc8c0cdd5d03803ee081cea2e304ce/image-15.jpg)
Kinetics of enzyme catalysis and the Michaelis–Menten equation: � Description of v–[S] curves: or � Constants a and b have chemical meaning: a: as [S] increases, v saturates so that a further increase in [S] does not accelerate the product formation any more. In this case [S] ≫ b, i. e. a = vmax. b: when b = [S], v = vmax/2 holds, so [S]1/2 = b. Leonor Michaelis and. Maud Leonora Menten (1912). 15
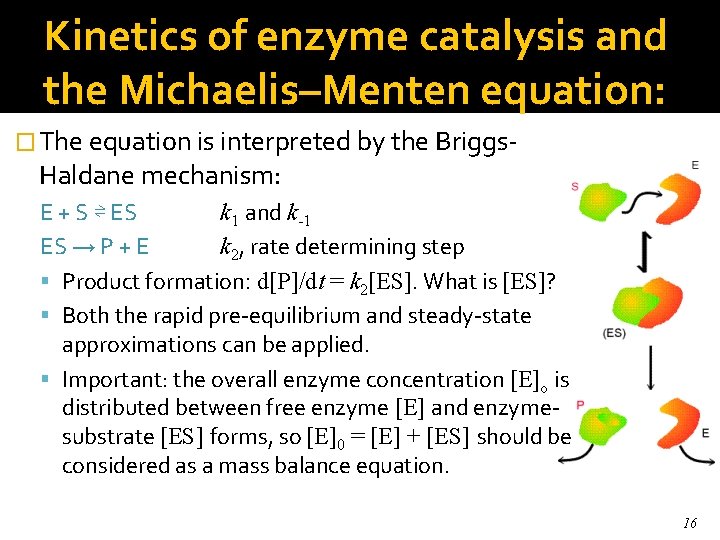
Kinetics of enzyme catalysis and the Michaelis–Menten equation: � The equation is interpreted by the Briggs- Haldane mechanism: E + S ⇌ ES k 1 and k-1 ES → P + E k 2, rate determining step Product formation: d[P]/dt = k 2[ES]. What is [ES]? Both the rapid pre-equilibrium and steady-state approximations can be applied. Important: the overall enzyme concentration [E]o is distributed between free enzyme [E] and enzymesubstrate [ES] forms, so [E]0 = [E] + [ES] should be considered as a mass balance equation. 16
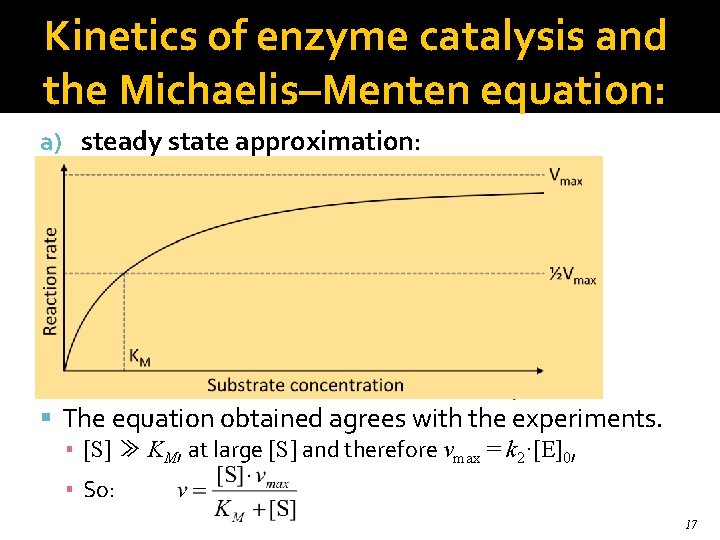
Kinetics of enzyme catalysis and the Michaelis–Menten equation: a) steady state approximation: d. ES/dt = k 1·E·S – k-1·ES – k 2·ES = 0 So: KM is the Michaelis constant: . The equation obtained agrees with the experiments. ▪ [S] ≫ KM, at large [S] and therefore vmax = k 2·[E]0, ▪ So: 17
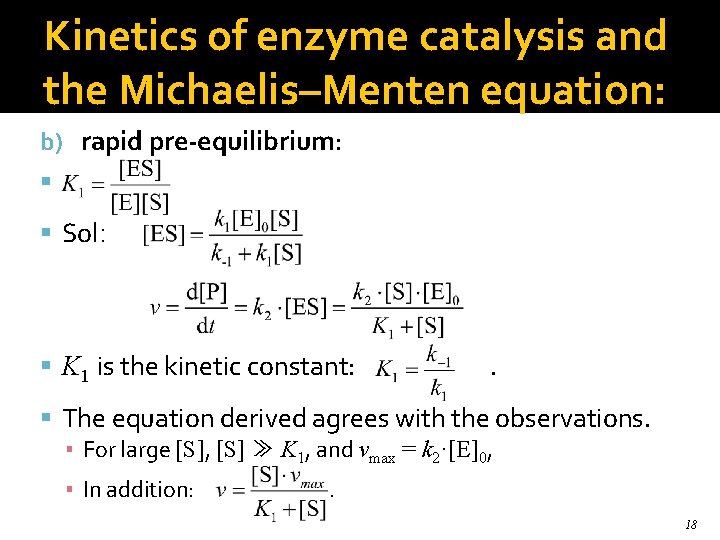
Kinetics of enzyme catalysis and the Michaelis–Menten equation: b) rapid pre-equilibrium: Sol: K 1 is the kinetic constant: . The equation derived agrees with the observations. ▪ For large [S], [S] ≫ K 1, and vmax = k 2·[E]0, ▪ In addition: . 18
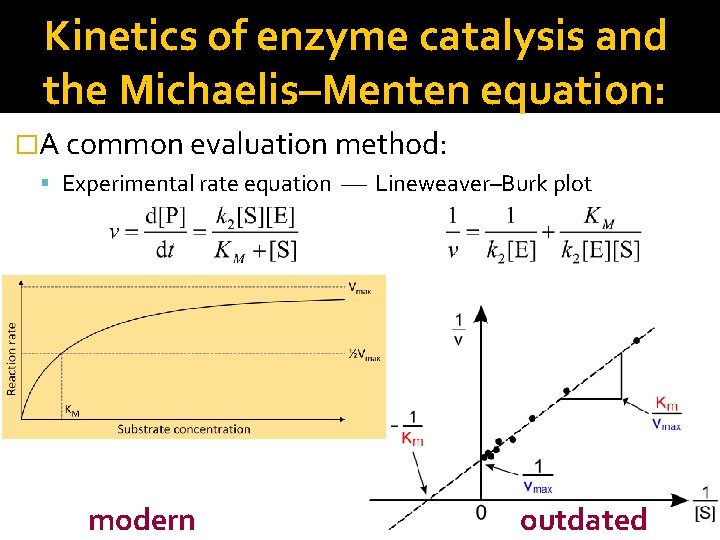
Kinetics of enzyme catalysis and the Michaelis–Menten equation: �A common evaluation method: Experimental rate equation Lineweaver–Burk plot modern outdated
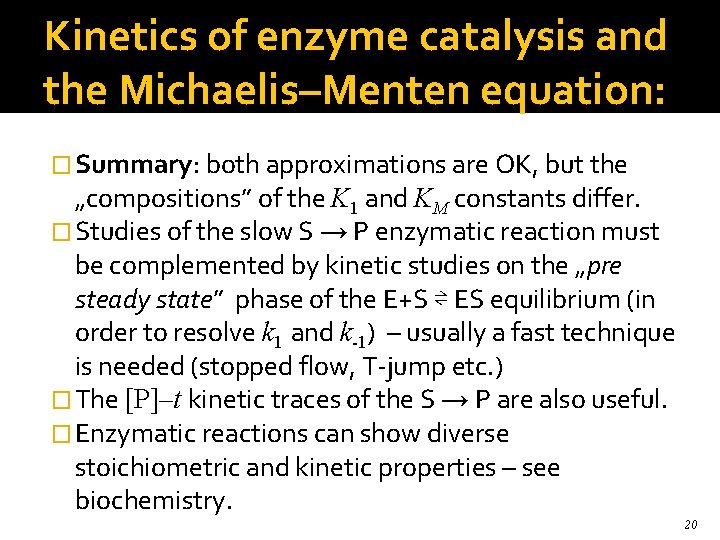
Kinetics of enzyme catalysis and the Michaelis–Menten equation: � Summary: both approximations are OK, but the „compositions” of the K 1 and KM constants differ. � Studies of the slow S → P enzymatic reaction must be complemented by kinetic studies on the „pre steady state” phase of the E+S ⇌ ES equilibrium (in order to resolve k 1 and k-1) – usually a fast technique is needed (stopped flow, T-jump etc. ) � The [P]–t kinetic traces of the S → P are also useful. � Enzymatic reactions can show diverse stoichiometric and kinetic properties – see biochemistry. 20
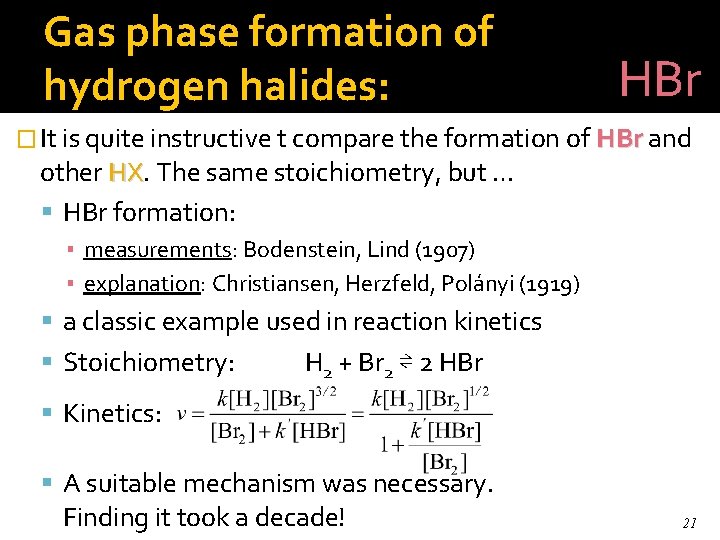
Gas phase formation of hydrogen halides: HBr � It is quite instructive t compare the formation of HBr and other HX. HX The same stoichiometry, but … HBr formation: ▪ measurements: Bodenstein, Lind (1907) ▪ explanation: Christiansen, Herzfeld, Polányi (1919) a classic example used in reaction kinetics Stoichiometry: H 2 + Br 2 ⇌ 2 HBr Kinetics: A suitable mechanism was necessary. Finding it took a decade! 21
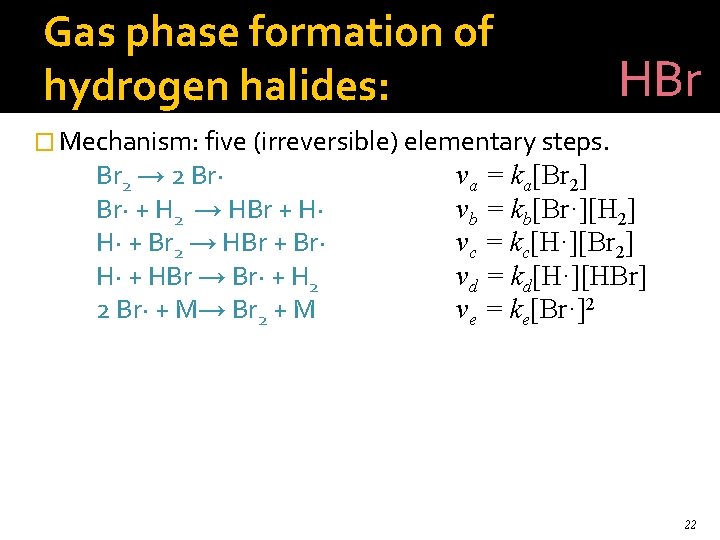
Gas phase formation of hydrogen halides: HBr � Mechanism: five (irreversible) elementary steps. Br 2 → 2 Br· + H 2 → HBr + H· H· + Br 2 → HBr + Br· H· + HBr → Br· + H 2 2 Br· + M→ Br 2 + M va = ka[Br 2] vb = kb[Br·][H 2] vc = kc[H·][Br 2] vd = kd[H·][HBr] ve = ke[Br·]2 22
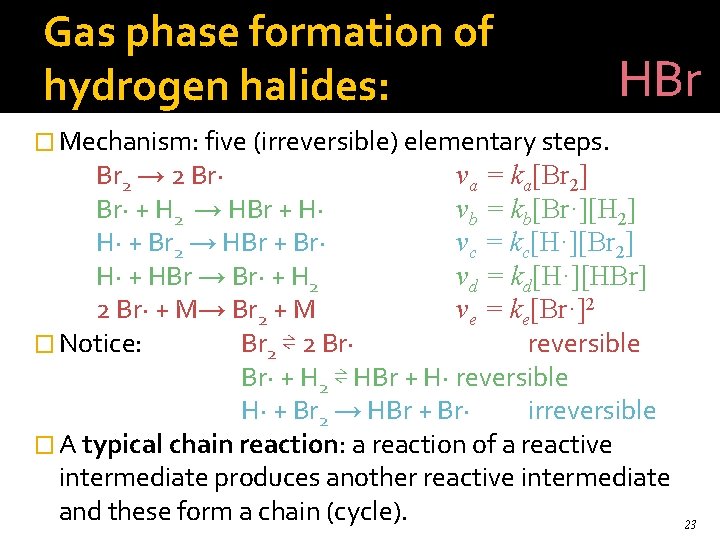
Gas phase formation of hydrogen halides: HBr � Mechanism: five (irreversible) elementary steps. Br 2 → 2 Br· va = ka[Br 2] Br· + H 2 → HBr + H· vb = kb[Br·][H 2] H· + Br 2 → HBr + Br· vc = kc[H·][Br 2] H· + HBr → Br· + H 2 vd = kd[H·][HBr] 2 Br· + M→ Br 2 + M ve = ke[Br·]2 � Notice: Br 2 ⇌ 2 Br· reversible Br· + H 2 ⇌ HBr + H· reversible H· + Br 2 → HBr + Br· irreversible � A typical chain reaction: a reaction of a reactive intermediate produces another reactive intermediate and these form a chain (cycle). 23
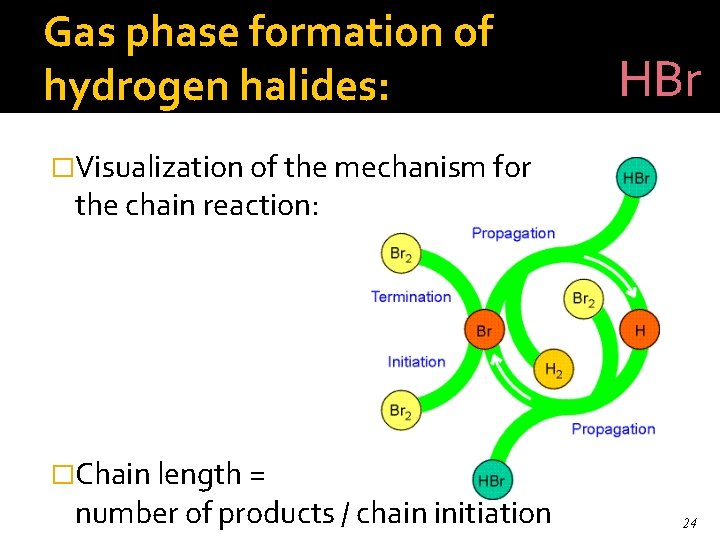
Gas phase formation of hydrogen halides: HBr �Visualization of the mechanism for the chain reaction: �Chain length = number of products / chain initiation 24
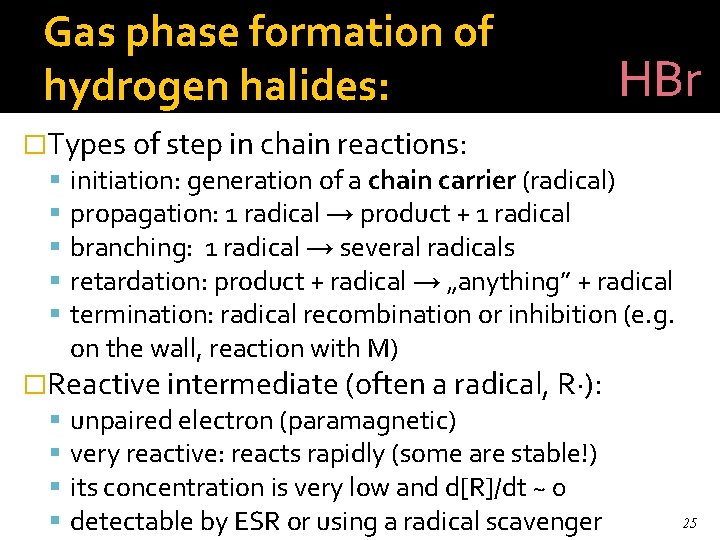
Gas phase formation of hydrogen halides: HBr �Types of step in chain reactions: initiation: generation of a chain carrier (radical) propagation: 1 radical → product + 1 radical branching: 1 radical → several radicals retardation: product + radical → „anything” + radical termination: radical recombination or inhibition (e. g. on the wall, reaction with M) �Reactive intermediate (often a radical, R·): unpaired electron (paramagnetic) very reactive: reacts rapidly (some are stable!) its concentration is very low and d[R]/dt ~ 0 detectable by ESR or using a radical scavenger 25
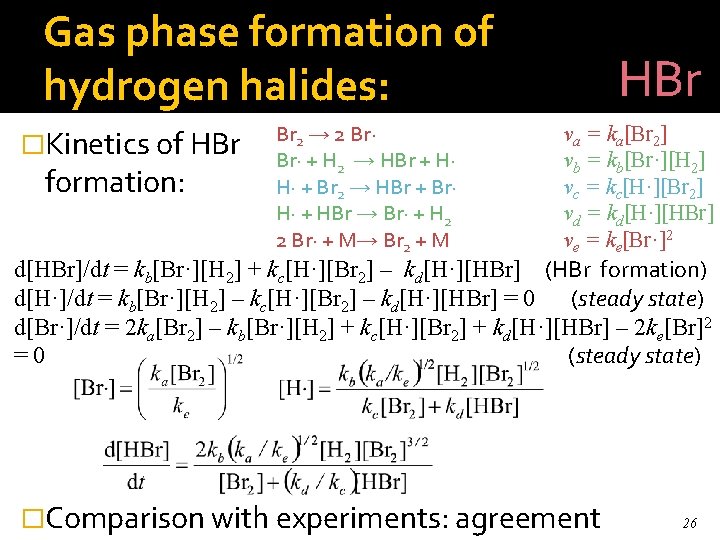
Gas phase formation of hydrogen halides: �Kinetics of HBr formation: Br 2 → 2 Br· + H 2 → HBr + H· H· + Br 2 → HBr + Br· H· + HBr → Br· + H 2 2 Br· + M→ Br 2 + M HBr va = ka[Br 2] vb = kb[Br·][H 2] vc = kc[H·][Br 2] vd = kd[H·][HBr] ve = ke[Br·]2 d[HBr]/dt = kb[Br·][H 2] + kc[H·][Br 2] – kd[H·][HBr] (HBr formation) d[H·]/dt = kb[Br·][H 2] – kc[H·][Br 2] – kd[H·][HBr] = 0 (steady state) d[Br·]/dt = 2 ka[Br 2] – kb[Br·][H 2] + kc[H·][Br 2] + kd[H·][HBr] – 2 ke[Br]2 =0 (steady state) �Comparison with experiments: agreement 26
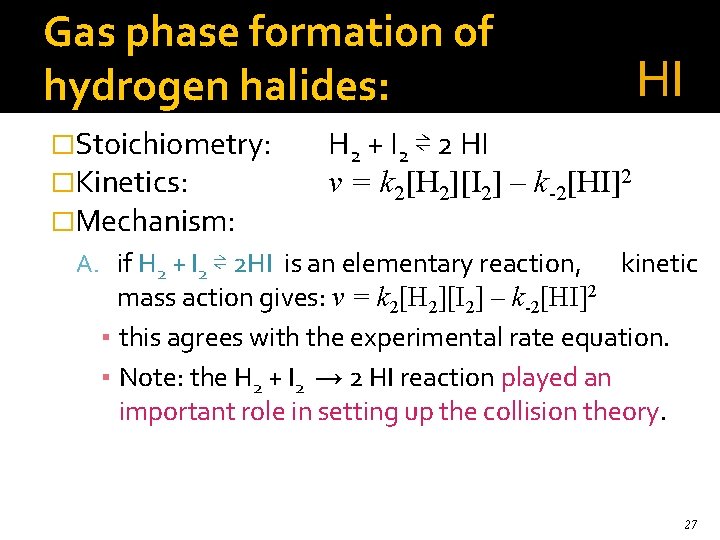
Gas phase formation of hydrogen halides: �Stoichiometry: �Kinetics: �Mechanism: HI H 2 + I 2 ⇌ 2 HI v = k 2[H 2][I 2] – k-2[HI]2 A. if H 2 + I 2 ⇌ 2 HI is an elementary reaction, kinetic mass action gives: v = k 2[H 2][I 2] – k-2[HI]2 ▪ this agrees with the experimental rate equation. ▪ Note: the H 2 + I 2 → 2 HI reaction played an important role in setting up the collision theory. 27
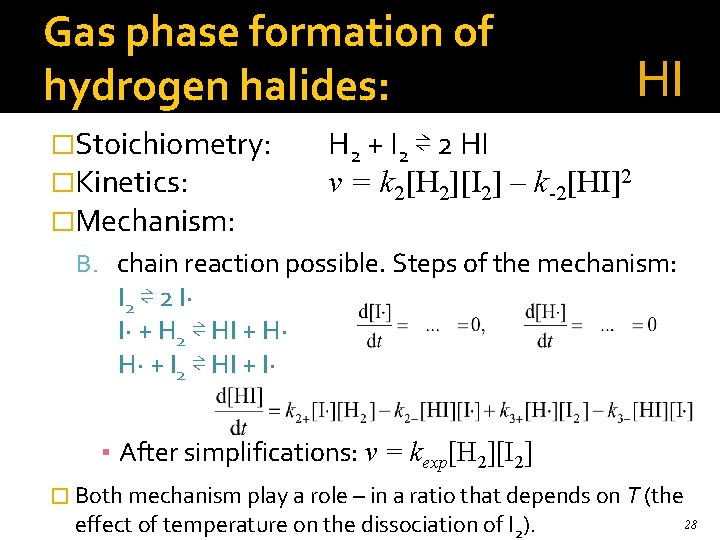
Gas phase formation of hydrogen halides: �Stoichiometry: �Kinetics: �Mechanism: HI H 2 + I 2 ⇌ 2 HI v = k 2[H 2][I 2] – k-2[HI]2 B. chain reaction possible. Steps of the mechanism: I 2 ⇌ 2 I· I· + H 2 ⇌ HI + H· H· + I 2 ⇌ HI + I· ▪ After simplifications: v = kexp[H 2][I 2] � Both mechanism play a role – in a ratio that depends on T (the effect of temperature on the dissociation of I 2). 28
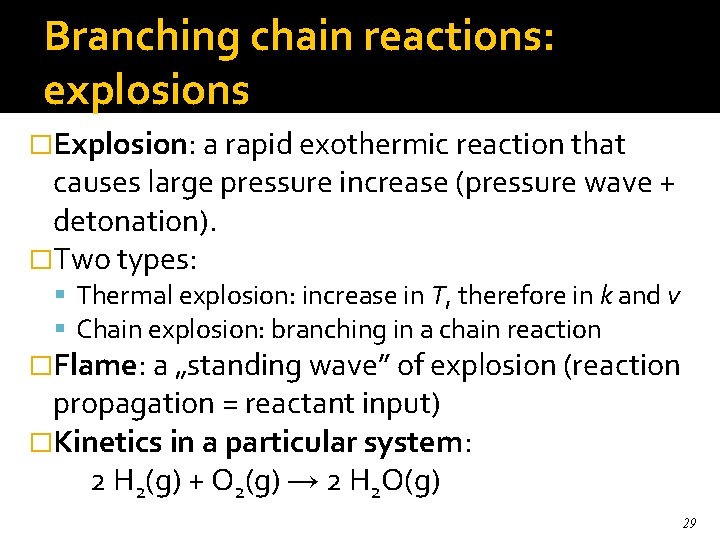
Branching chain reactions: explosions �Explosion: a rapid exothermic reaction that causes large pressure increase (pressure wave + detonation). �Two types: Thermal explosion: increase in T, therefore in k and v Chain explosion: branching in a chain reaction �Flame: a „standing wave” of explosion (reaction propagation = reactant input) �Kinetics in a particular system: 2 H 2(g) + O 2(g) → 2 H 2 O(g) 29
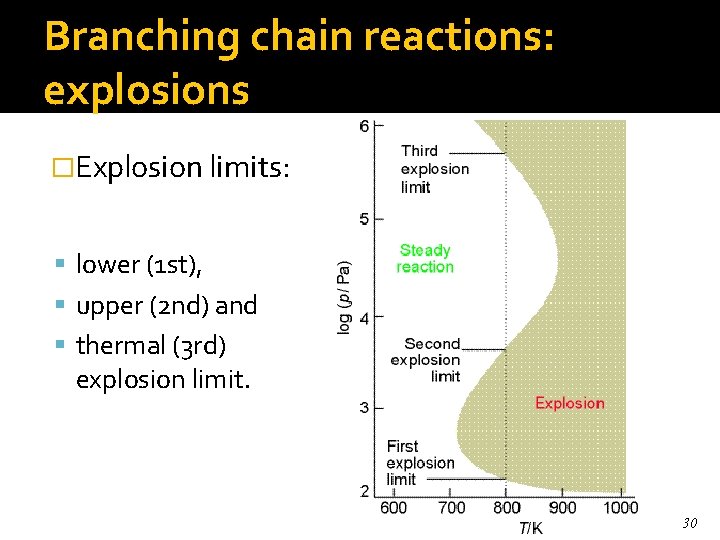
Branching chain reactions: explosions �Explosion limits: lower (1 st), upper (2 nd) and thermal (3 rd) explosion limit. 30
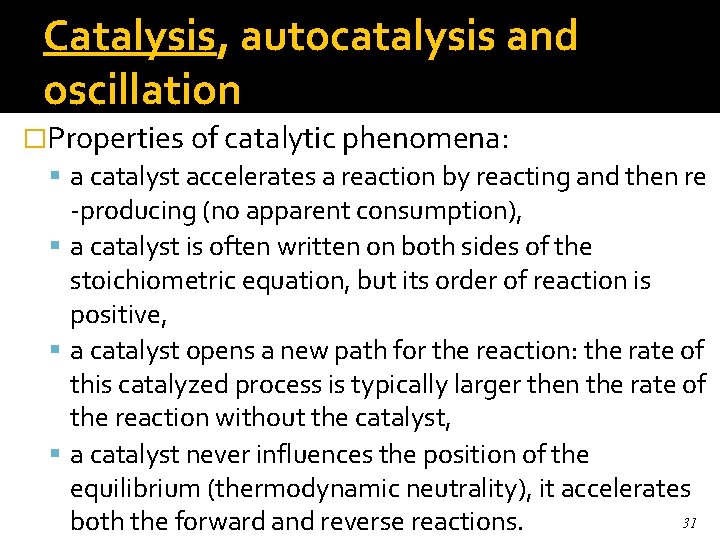
Catalysis, autocatalysis and oscillation �Properties of catalytic phenomena: a catalyst accelerates a reaction by reacting and then re -producing (no apparent consumption), a catalyst is often written on both sides of the stoichiometric equation, but its order of reaction is positive, a catalyst opens a new path for the reaction: the rate of this catalyzed process is typically larger then the rate of the reaction without the catalyst, a catalyst never influences the position of the equilibrium (thermodynamic neutrality), it accelerates 31 both the forward and reverse reactions.
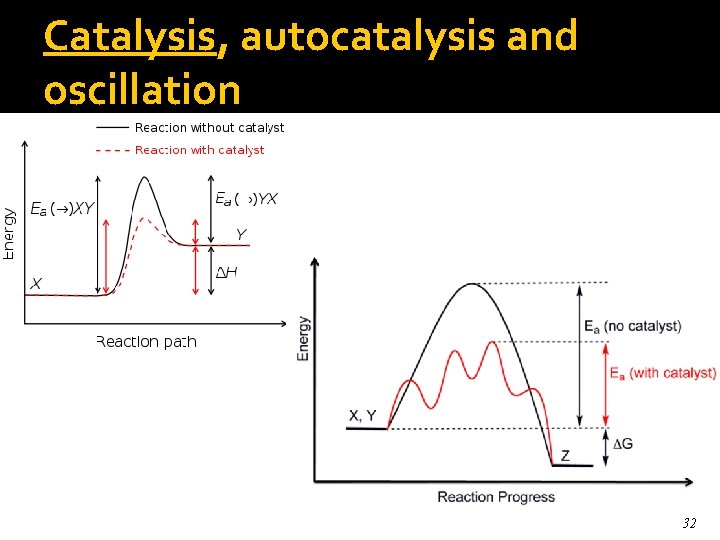
Catalysis, autocatalysis and oscillation 32
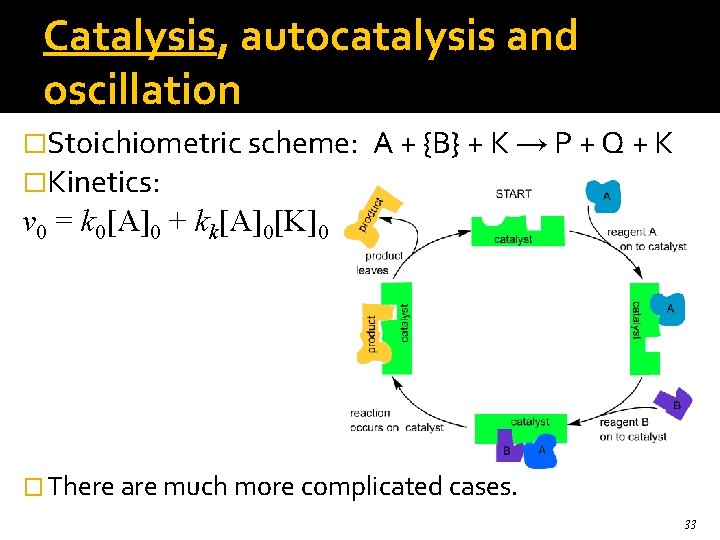
Catalysis, autocatalysis and oscillation �Stoichiometric scheme: �Kinetics: A + {B} + K → P + Q + K v 0 = k 0[A]0 + kk[A]0[K]0 � There are much more complicated cases. 33
![Catalysis autocatalysis and oscillation Autocatalysis Stoichiometry A Kinetics v P k 0A Catalysis, autocatalysis and oscillation �Autocatalysis: �Stoichiometry: A �Kinetics: v →P = k 0[A] +](https://slidetodoc.com/presentation_image_h2/8dfc8c0cdd5d03803ee081cea2e304ce/image-34.jpg)
Catalysis, autocatalysis and oscillation �Autocatalysis: �Stoichiometry: A �Kinetics: v →P = k 0[A] + kak[A][P] �Typical autocatalytic trace: S-shaped induction period point of inflection: vmax �E. g. : Mn. O 4 - + oxalate (Mn 2+ catalysis) ▪ https: //www. youtube. com/watch? v=i. Jiy 38 s. Ex. PE � Soai reaction (chiral autocatalysis) 34
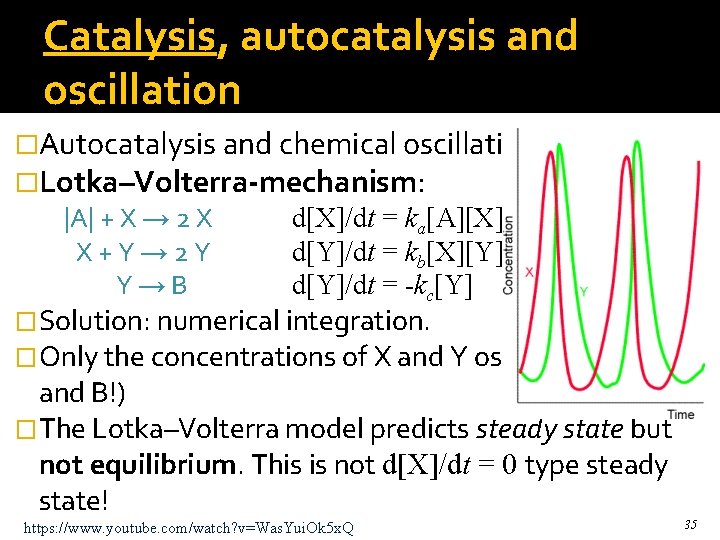
Catalysis, autocatalysis and oscillation �Autocatalysis and chemical oscillation. �Lotka–Volterra-mechanism: |A| + X → 2 X X+Y→ 2 Y Y→B d[X]/dt = ka[A][X] d[Y]/dt = kb[X][Y] d[Y]/dt = -kc[Y] �Solution: numerical integration. �Only the concentrations of X and Y oscillate (not A and B!) �The Lotka–Volterra model predicts steady state but not equilibrium. This is not d[X]/dt = 0 type steady state! https: //www. youtube. com/watch? v=Was. Yui. Ok 5 x. Q 35
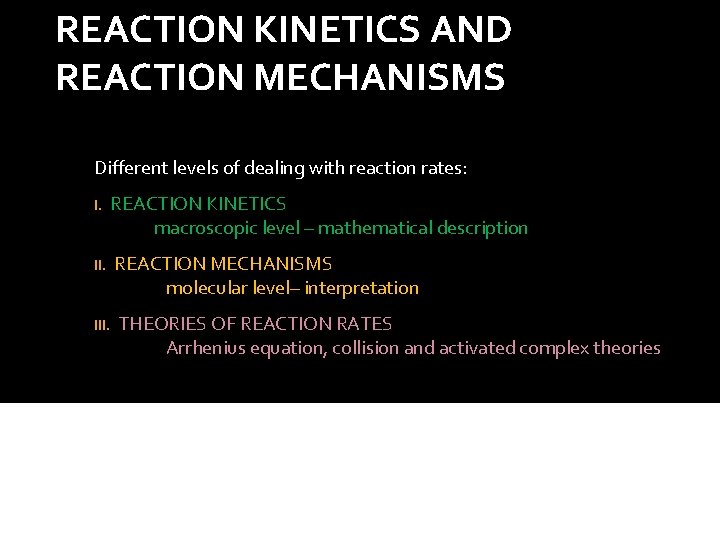
REACTION KINETICS AND REACTION MECHANISMS Different levels of dealing with reaction rates: I. REACTION KINETICS macroscopic level – mathematical description II. REACTION MECHANISMS molecular level– interpretation III. THEORIES OF REACTION RATES Arrhenius equation, collision and activated complex theories 36
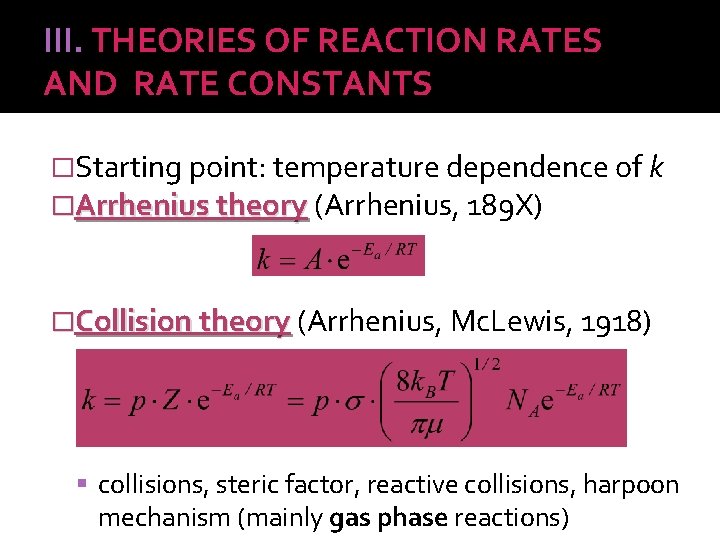
III. THEORIES OF REACTION RATES AND RATE CONSTANTS �Starting point: temperature dependence of k �Arrhenius theory (Arrhenius, 189 X) �Collision theory (Arrhenius, Mc. Lewis, 1918) collisions, steric factor, reactive collisions, harpoon mechanism (mainly gas phase reactions)
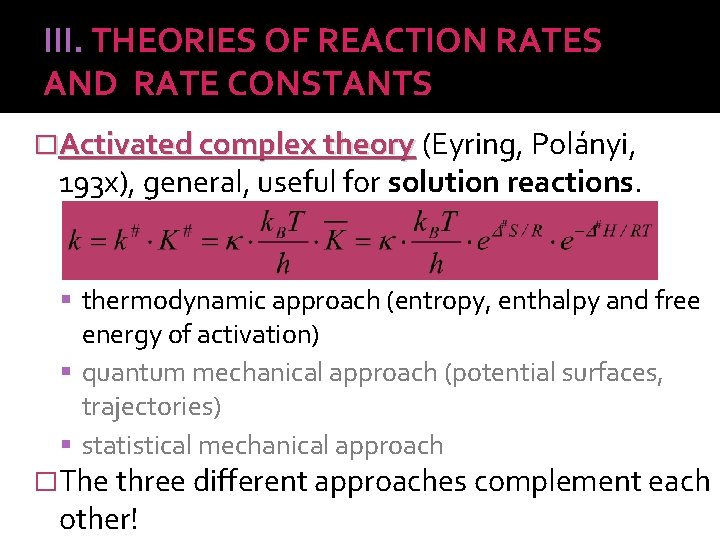
III. THEORIES OF REACTION RATES AND RATE CONSTANTS �Activated complex theory (Eyring, Polányi, 193 x), general, useful for solution reactions. thermodynamic approach (entropy, enthalpy and free energy of activation) quantum mechanical approach (potential surfaces, trajectories) statistical mechanical approach �The three different approaches complement each other!
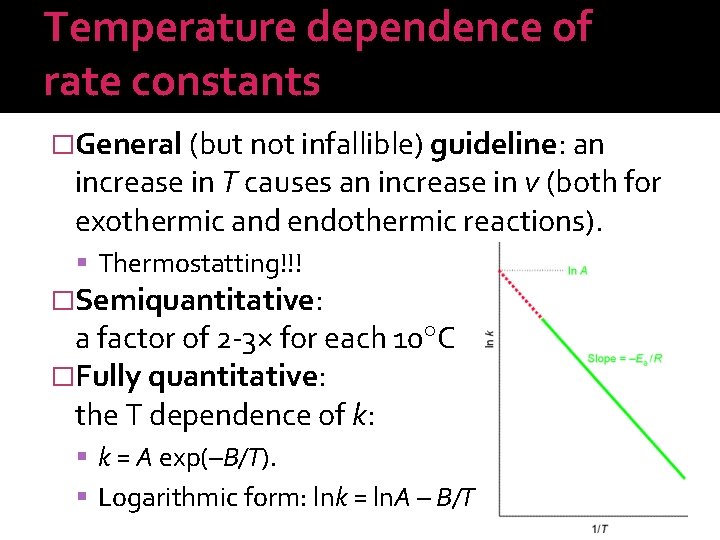
Temperature dependence of rate constants �General (but not infallible) guideline: an increase in T causes an increase in v (both for exothermic and endothermic reactions). Thermostatting!!! �Semiquantitative: a factor of 2 -3× for each 10 C �Fully quantitative: the T dependence of k: k = A exp(–B/T). Logarithmic form: lnk = ln. A – B/T
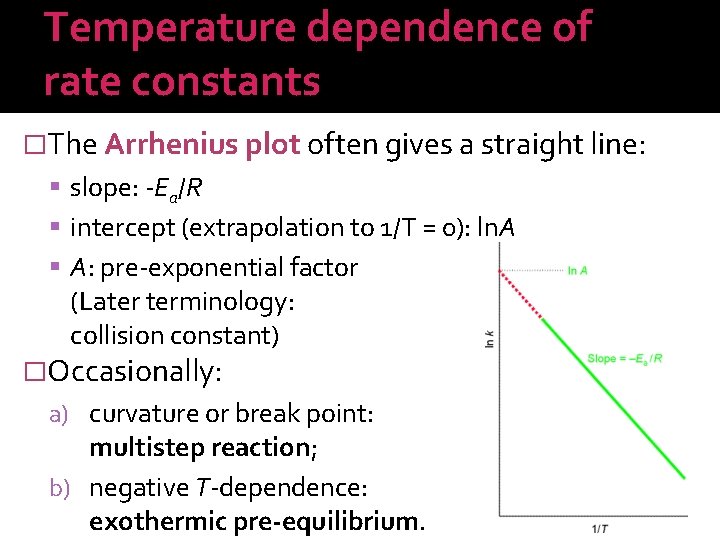
Temperature dependence of rate constants �The Arrhenius plot often gives a straight line: slope: -Ea/R intercept (extrapolation to 1/T = 0): ln. A A: pre-exponential factor (Later terminology: collision constant) �Occasionally: a) curvature or break point: multistep reaction; b) negative T-dependence: exothermic pre-equilibrium.
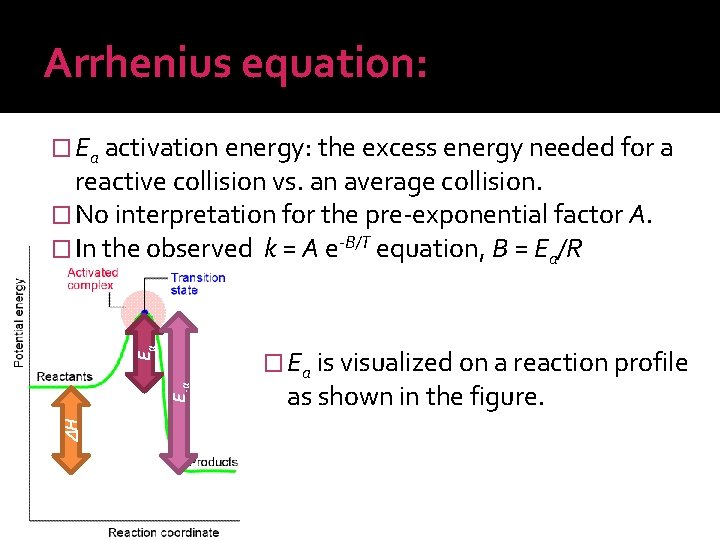
Arrhenius equation: � Ea activation energy: the excess energy needed for a H E-a Ea reactive collision vs. an average collision. � No interpretation for the pre-exponential factor A. � In the observed k = A e-B/T equation, B = Ea/R � Ea is visualized on a reaction profile as shown in the figure.
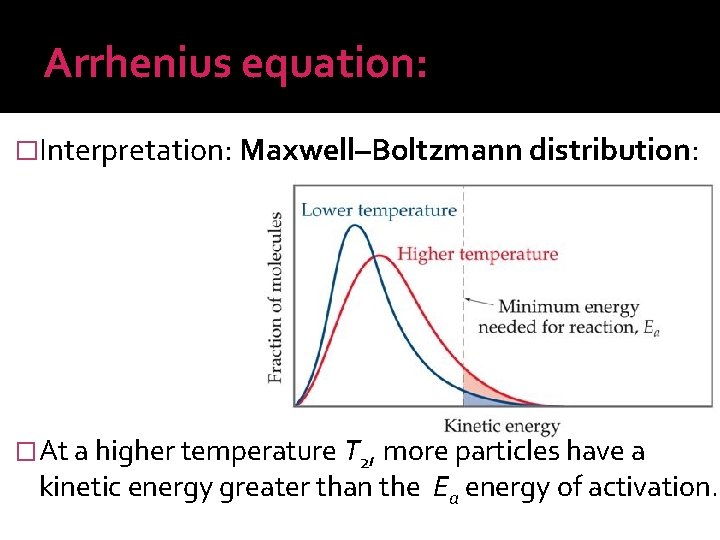
Arrhenius equation: �Interpretation: Maxwell–Boltzmann distribution: �At a higher temperature T 2, more particles have a kinetic energy greater than the Ea energy of activation.
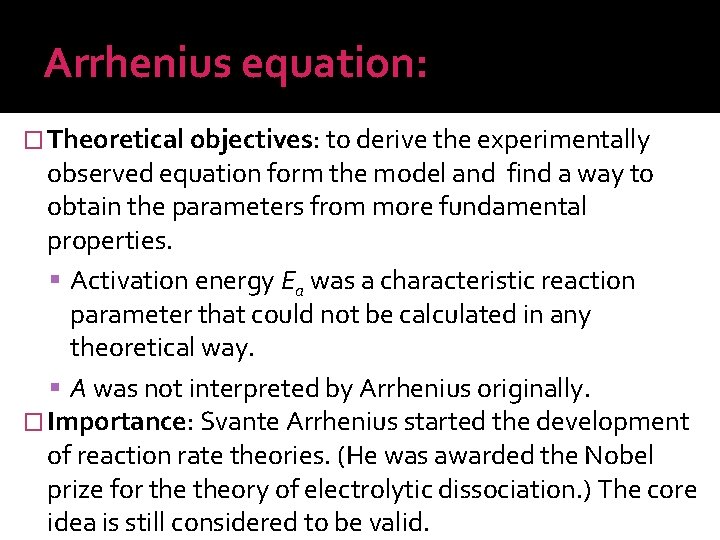
Arrhenius equation: � Theoretical objectives: to derive the experimentally observed equation form the model and find a way to obtain the parameters from more fundamental properties. Activation energy Ea was a characteristic reaction parameter that could not be calculated in any theoretical way. A was not interpreted by Arrhenius originally. � Importance: Svante Arrhenius started the development of reaction rate theories. (He was awarded the Nobel prize for theory of electrolytic dissociation. ) The core idea is still considered to be valid.
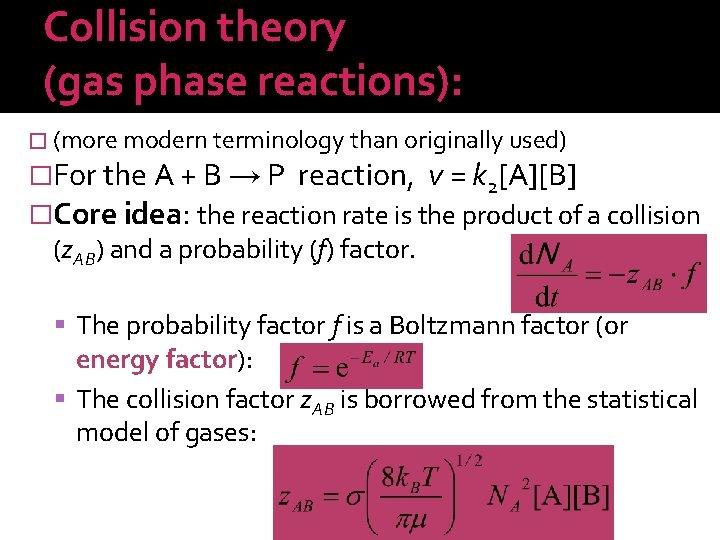
Collision theory (gas phase reactions): � (more modern terminology than originally used) �For the A + B → P reaction, v = k 2[A][B] �Core idea: the reaction rate is the product of a collision (z. AB) and a probability (f) factor. The probability factor f is a Boltzmann factor (or energy factor): The collision factor z. AB is borrowed from the statistical model of gases:
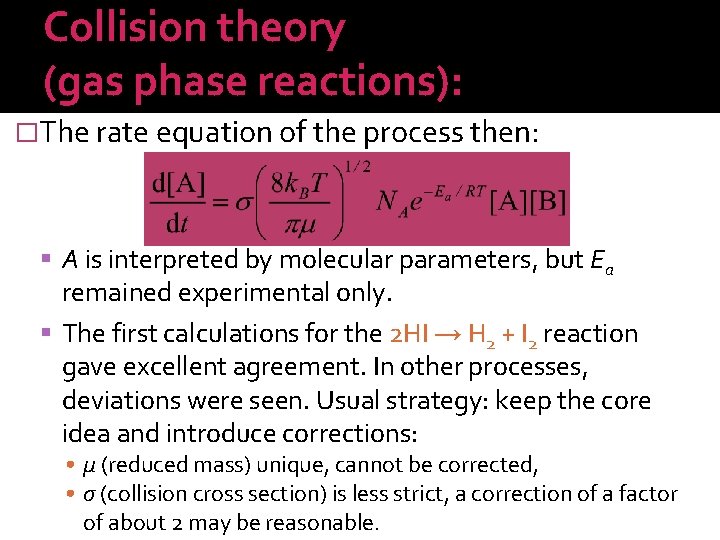
Collision theory (gas phase reactions): �The rate equation of the process then: A is interpreted by molecular parameters, but Ea remained experimental only. The first calculations for the 2 HI → H 2 + I 2 reaction gave excellent agreement. In other processes, deviations were seen. Usual strategy: keep the core idea and introduce corrections: • μ (reduced mass) unique, cannot be corrected, • σ (collision cross section) is less strict, a correction of a factor of about 2 may be reasonable.
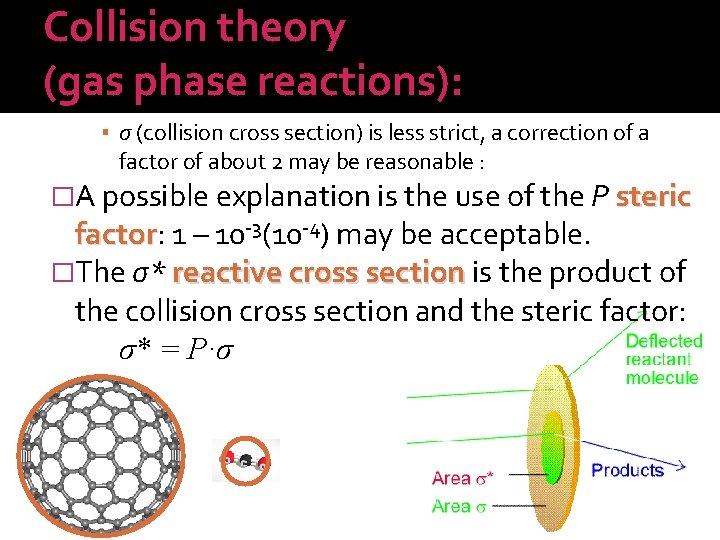
Collision theory (gas phase reactions): ▪ σ (collision cross section) is less strict, a correction of a factor of about 2 may be reasonable : �A possible explanation is the use of the P steric factor: factor 1 – 10 -3(10 -4) may be acceptable. �The σ* reactive cross section is the product of the collision cross section and the steric factor: σ* = P·σ
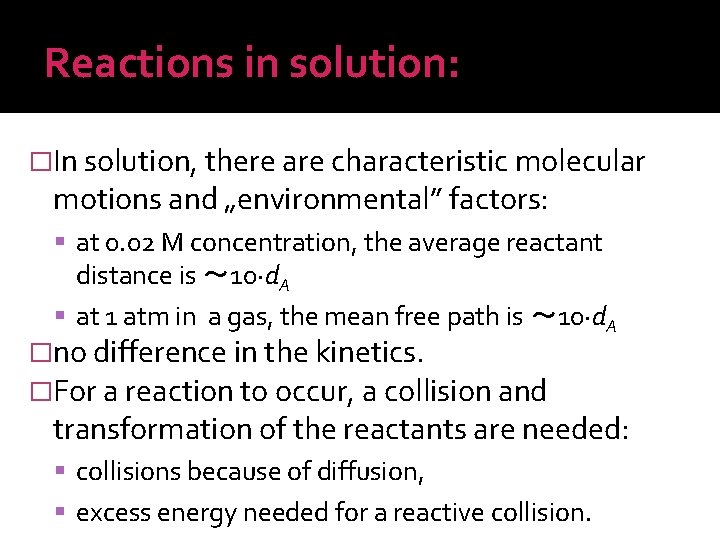
Reactions in solution: �In solution, there are characteristic molecular motions and „environmental” factors: at 0. 02 M concentration, the average reactant distance is 〜 10·d. A at 1 atm in a gas, the mean free path is 〜 10·d. A �no difference in the kinetics. �For a reaction to occur, a collision and transformation of the reactants are needed: collisions because of diffusion, excess energy needed for a reactive collision.
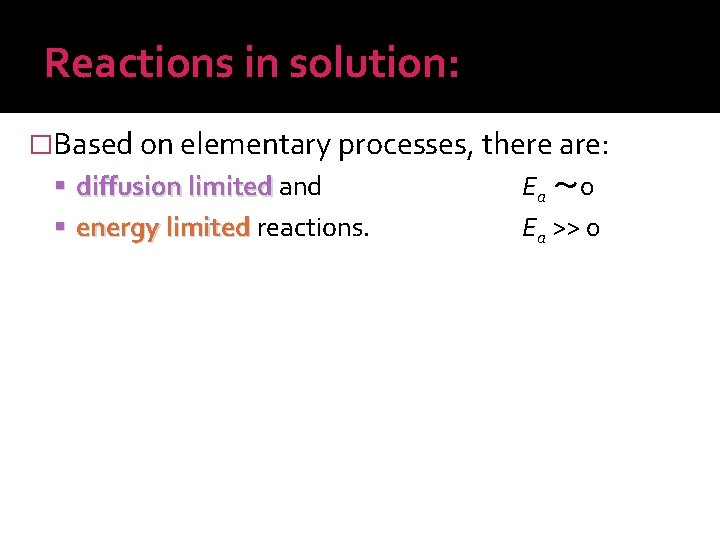
Reactions in solution: �Based on elementary processes, there are: diffusion limited and energy limited reactions. Ea 〜 0 Ea >> 0
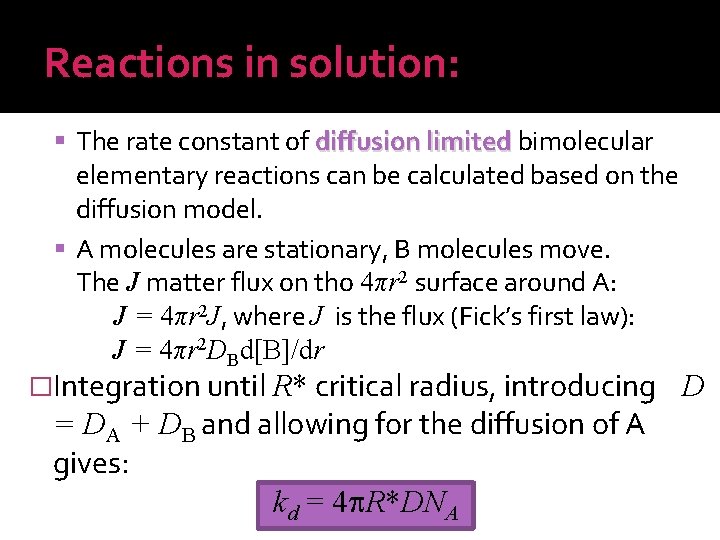
Reactions in solution: The rate constant of diffusion limited bimolecular elementary reactions can be calculated based on the diffusion model. A molecules are stationary, B molecules move. The J matter flux on tho 4πr 2 surface around A: J = 4πr 2 J, where J is the flux (Fick’s first law): J = 4πr 2 DBd[B]/dr �Integration until R* critical radius, introducing = DA + DB and allowing for the diffusion of A gives: kd = 4 R*DNA D
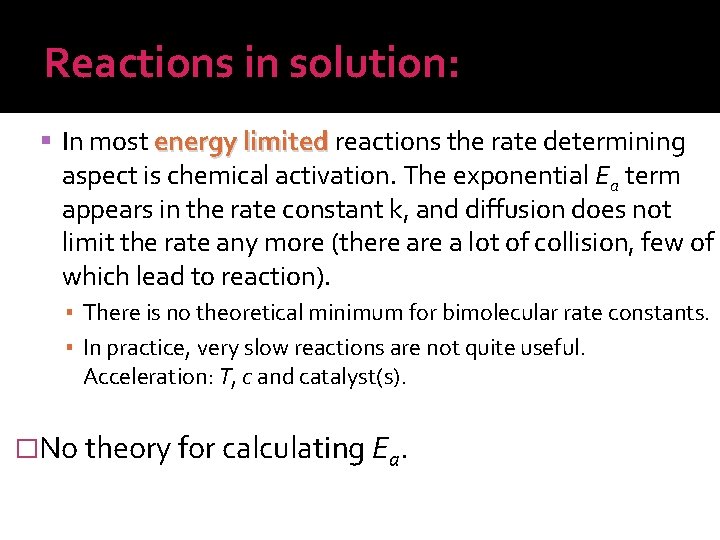
Reactions in solution: In most energy limited reactions the rate determining aspect is chemical activation. The exponential Ea term appears in the rate constant k, and diffusion does not limit the rate any more (there a lot of collision, few of which lead to reaction). ▪ There is no theoretical minimum for bimolecular rate constants. ▪ In practice, very slow reactions are not quite useful. Acceleration: T, c and catalyst(s). �No theory for calculating Ea.
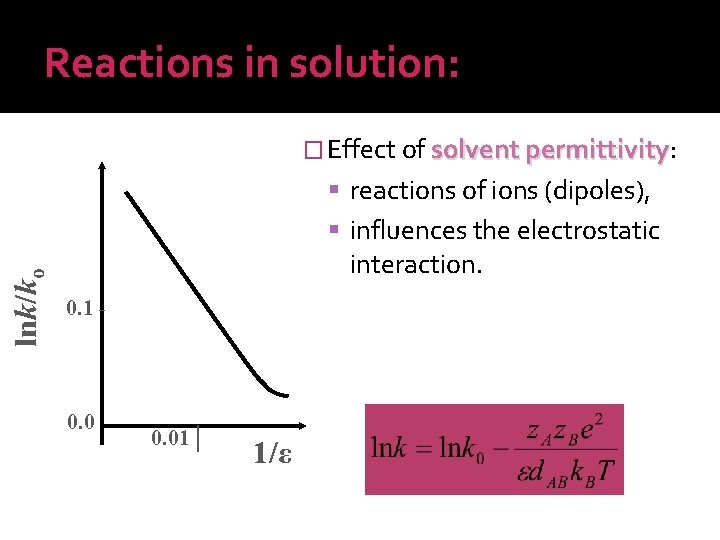
Reactions in solution: � Effect of solvent permittivity: permittivity reactions of ions (dipoles), lnk/ko influences the electrostatic interaction. 0. 1 - 0. 01│ 1/ε
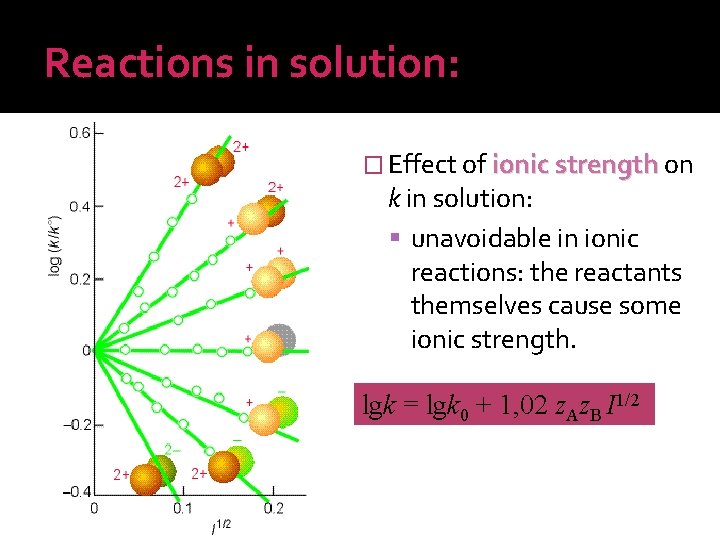
Reactions in solution: � Effect of ionic strength on k in solution: unavoidable in ionic reactions: the reactants themselves cause some ionic strength. lgk = lgk 0 + 1, 02 z. Az. B I 1/2
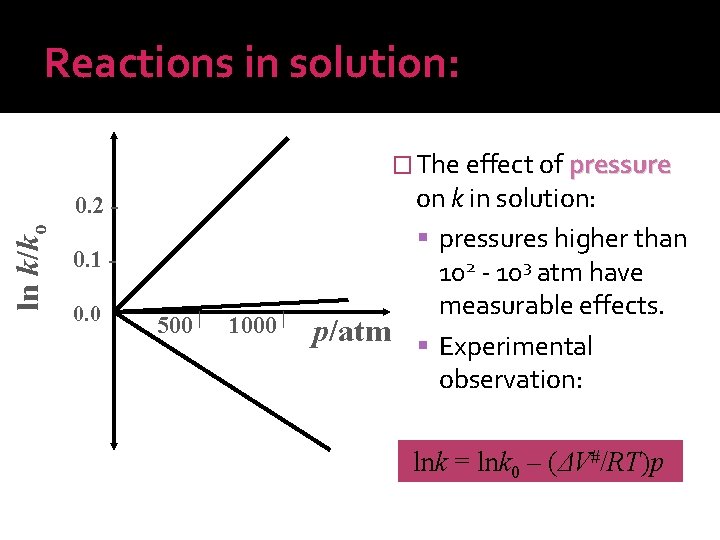
Reactions in solution: � The effect of pressure ln k/ko 0. 2 0. 1 0. 0 500 ▏ 1000 ▏ on k in solution: pressures higher than 102 - 103 atm have measurable effects. p/atm Experimental observation: lnk = lnk 0 – (ΔV#/RT)p
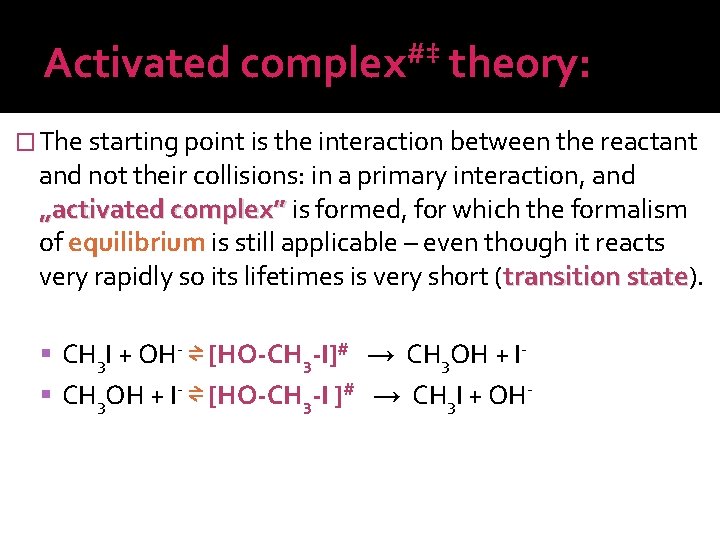
Activated #‡ complex theory: � The starting point is the interaction between the reactant and not their collisions: in a primary interaction, and „activated complex” is formed, for which the formalism of equilibrium is still applicable – even though it reacts very rapidly so its lifetimes is very short (transition state). state CH 3 I + OH- ⇌ [HO-CH 3 -I]# → CH 3 OH + I- ⇌ [HO-CH 3 -I ]# → CH 3 I + OH-
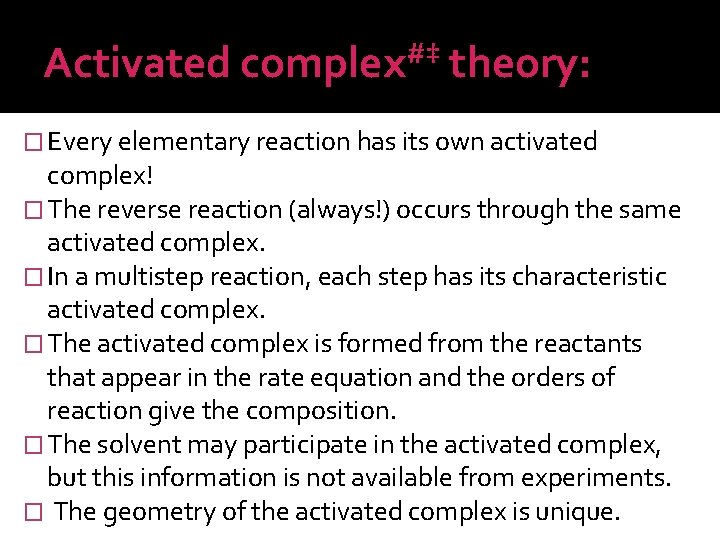
Activated #‡ complex theory: � Every elementary reaction has its own activated complex! � The reverse reaction (always!) occurs through the same activated complex. � In a multistep reaction, each step has its characteristic activated complex. � The activated complex is formed from the reactants that appear in the rate equation and the orders of reaction give the composition. � The solvent may participate in the activated complex, but this information is not available from experiments. � The geometry of the activated complex is unique.
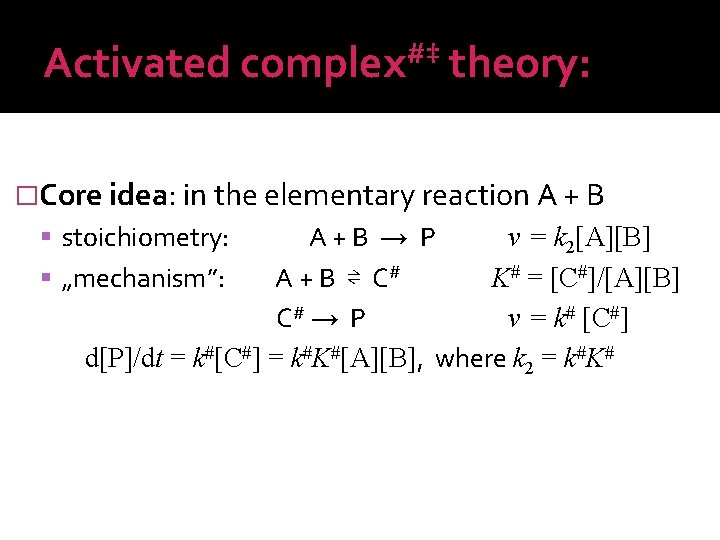
Activated #‡ complex theory: �Core idea: in the elementary reaction A + B stoichiometry: A+B → P v = k 2[A][B] „mechanism”: A + B ⇌ C# K# = [C#]/[A][B] C# → P v = k# [C#] d[P]/dt = k#[C#] = k#K#[A][B], where k 2 = k#K#
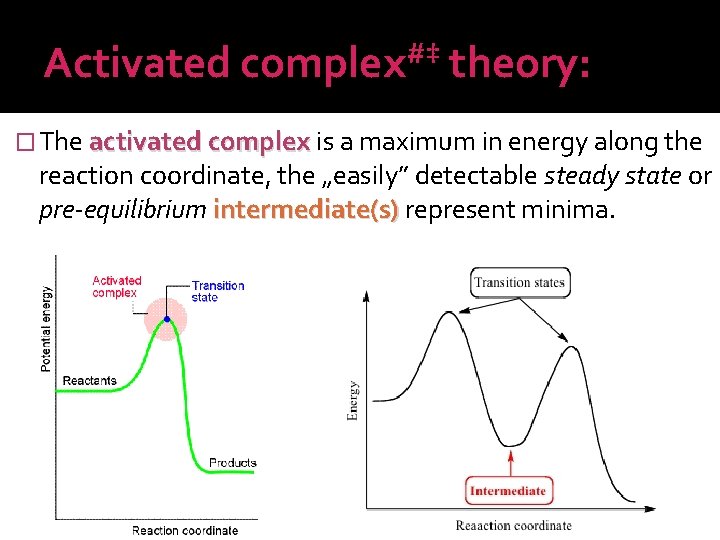
Activated #‡ complex theory: � The activated complex is a maximum in energy along the reaction coordinate, the „easily” detectable steady state or pre-equilibrium intermediate(s) represent minima.
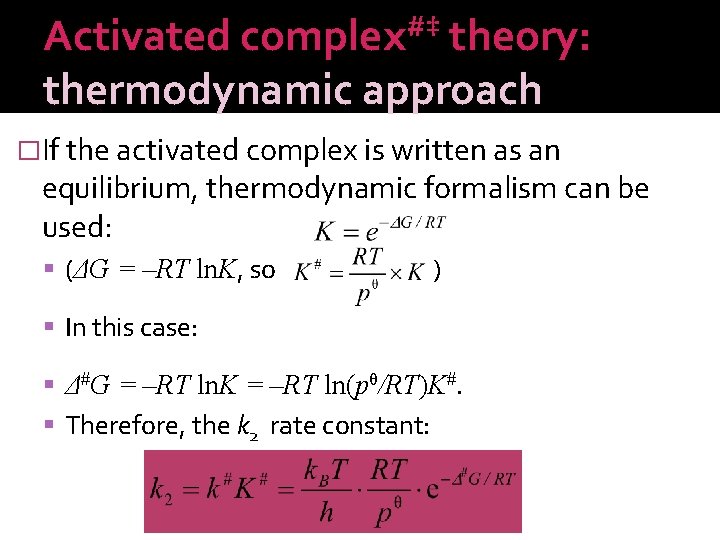
Activated complex#‡ theory: thermodynamic approach �If the activated complex is written as an equilibrium, thermodynamic formalism can be used: (ΔG = –RT ln. K, so ) In this case: Δ#G = –RT ln. K = –RT ln(pθ/RT)K#. Therefore, the k 2 rate constant:
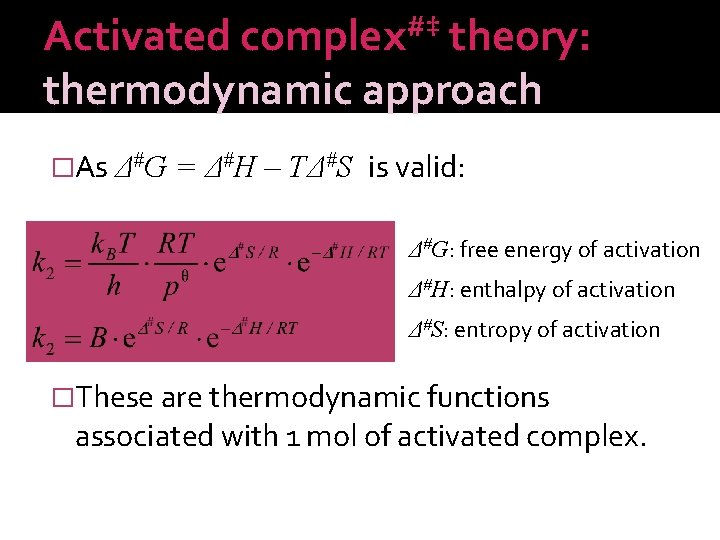
Activated complex#‡ theory: thermodynamic approach �As Δ#G = Δ#H – TΔ#S is valid: Δ#G: free energy of activation Δ#H: enthalpy of activation Δ#S: entropy of activation �These are thermodynamic functions associated with 1 mol of activated complex.
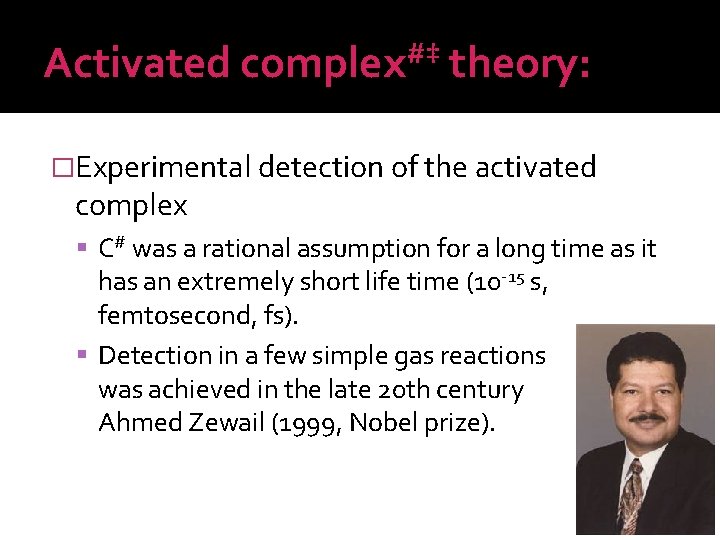
Activated #‡ complex theory: �Experimental detection of the activated complex C# was a rational assumption for a long time as it has an extremely short life time (10 -15 s, femtosecond, fs). Detection in a few simple gas reactions was achieved in the late 20 th century Ahmed Zewail (1999, Nobel prize).
Kinetics reaction
Different lifting mechanisms
Different file protection mechanism
Mechanisms of drug absorption
Different types of pop up mechanisms
The art of writing reasonable organic reaction mechanisms
Costa's house levels of questioning
Kinetics and equilibrium
Difference between 1st order and zero order kinetics
Difference between zero and first order kinetics
Kinetics of rigid bodies
Kinetics of a particle: force and acceleration
Kinetics of a particle: impulse and momentum
Leukocyte development kinetics and functions
Planar kinematics of a rigid body
Planar kinetics of a rigid body work and energy
Cell kinetics and fermenter design
Different sponsorship levels
Different levels of testing
Different court levels
Three redwood trees are kept at different humidity levels
Three levels of biodiversity
Top level management
What are the levels of ecological organization
Definition of chemical kinetics in chemistry
What are the factors affecting drug distribution
Rigid body kinetics
Collision theory of kinetics
Chemistry grade 11 unit 4 chemical kinetics
Kinetics flotation chemicals
Half life chemistry kinetics
Chemical kinetics definition
Motion of rigid body in three dimensions
Ap chemistry kinetics
Kinetics of crystal violet fading
Octet kinetics
Mixed inhibitor km and vmax
Pseudo first order equation
Kinetics of particles newton's second law
Chemical kinetics experiment
Dynafit kinetics
Enzyme kinetics
Data kinetics ltd
Kinetics 483
Applications of chemical kinetics
Rate of growth
Steady state approximation example
Kinetics is the branch of
Vampnets for deep learning of molecular kinetics
Ictahedron
Half-life formula
Why do different polymers have different properties?
Technicolor test
Sound will travel at different speeds in different mediums.
Examples of a medium in waves
What is cultural relativism
Different angle different story
Acids and bases have two different faces
Manufactured boards examples
No two people
Venn diagram different same different