Genome editing 1 Genome editing Genome editing or
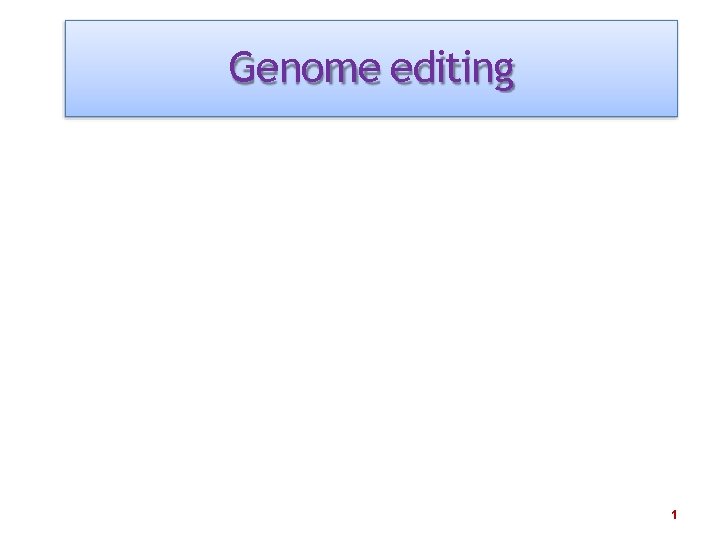
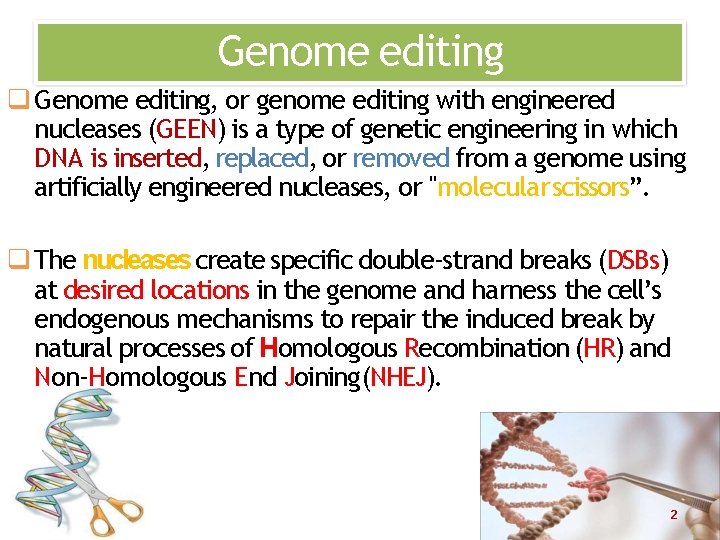
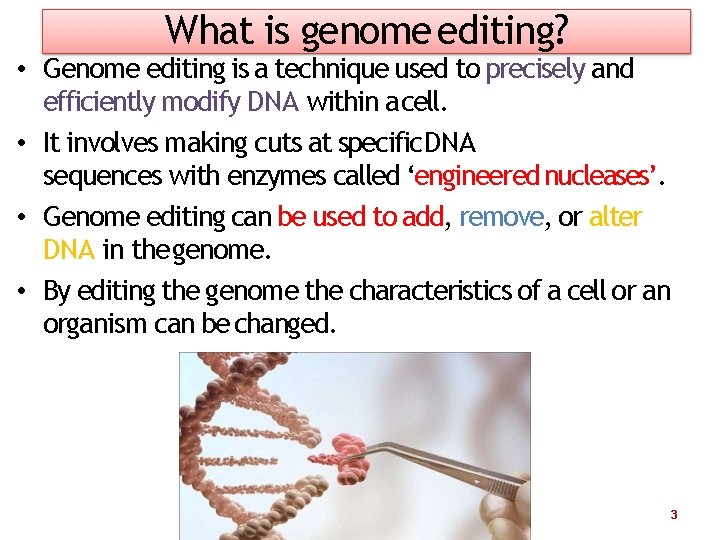
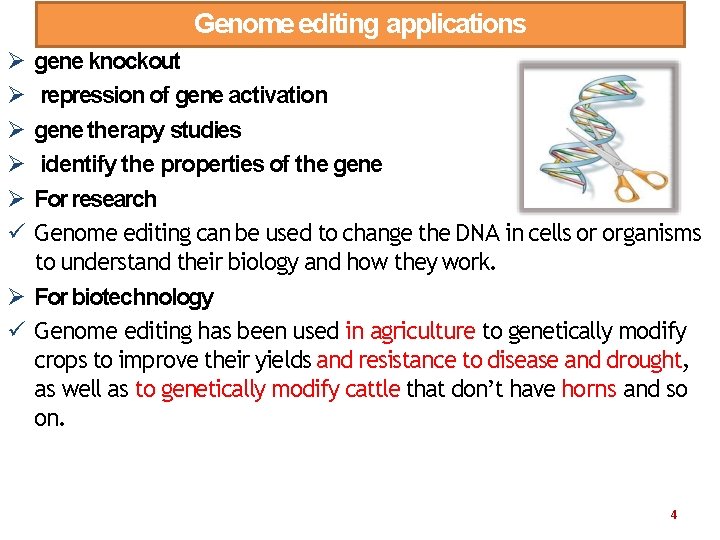
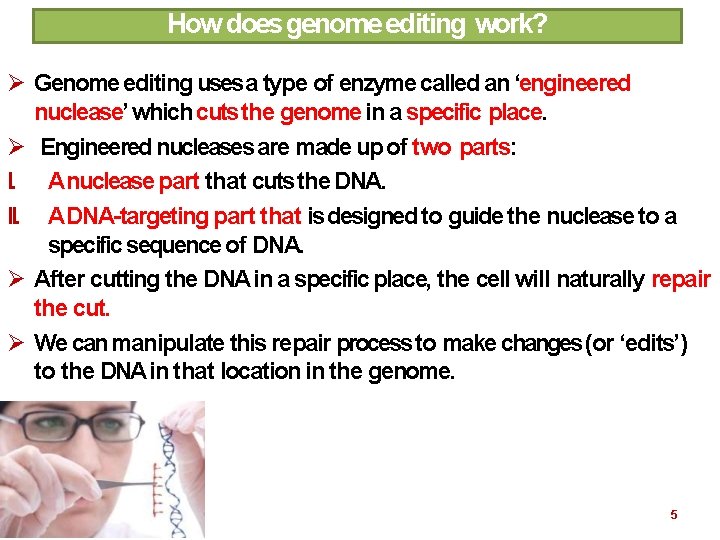
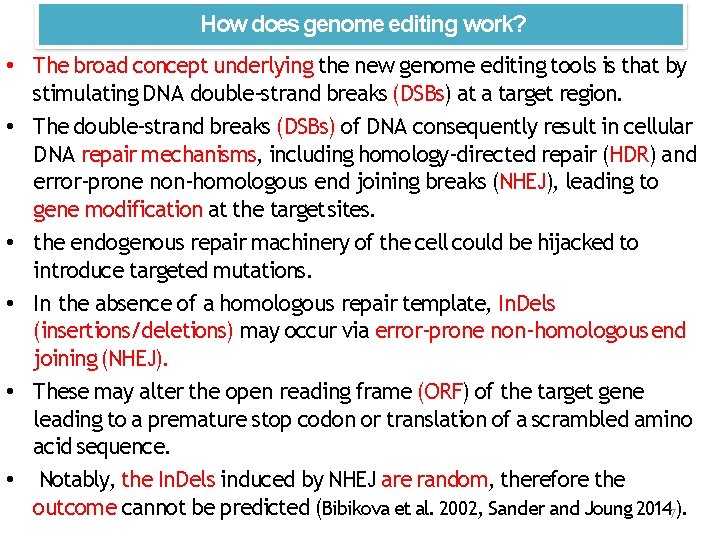
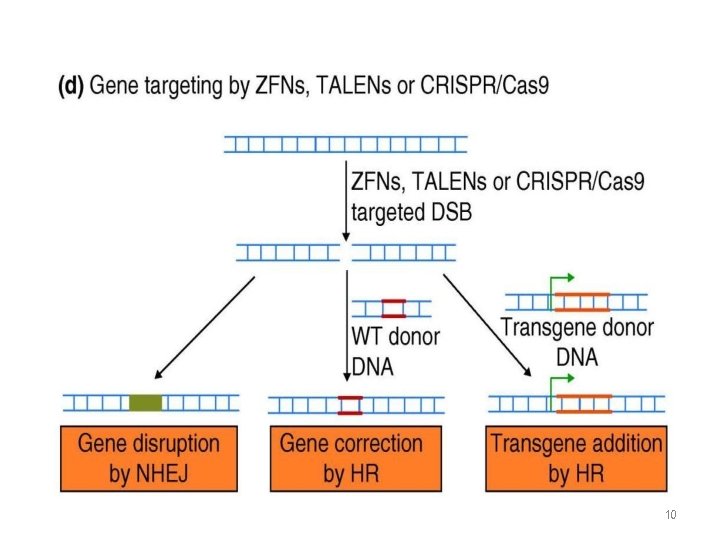
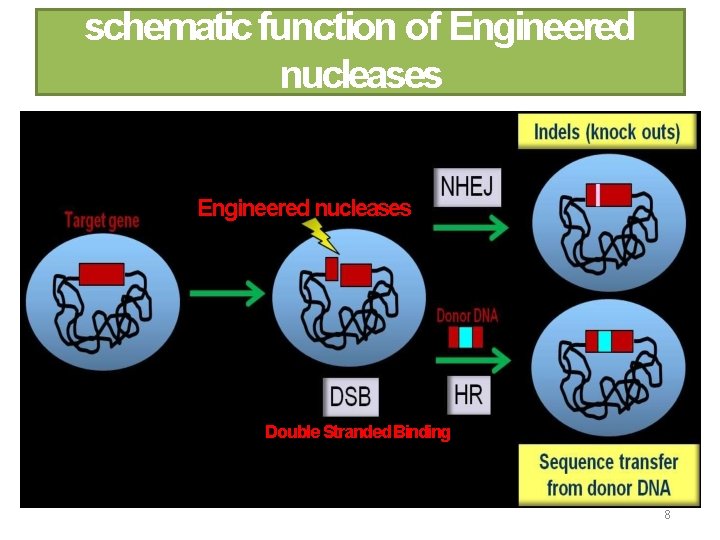
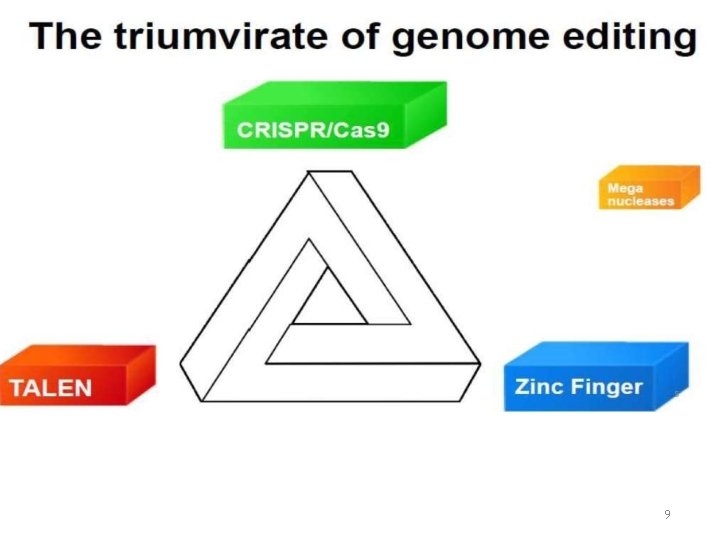
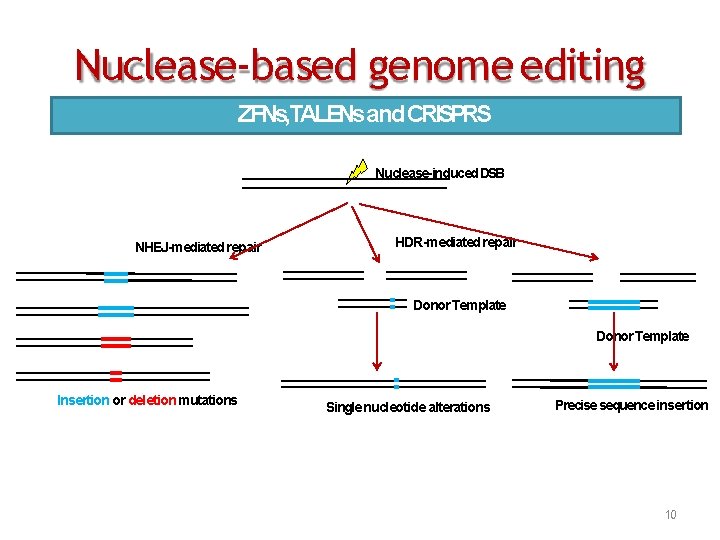
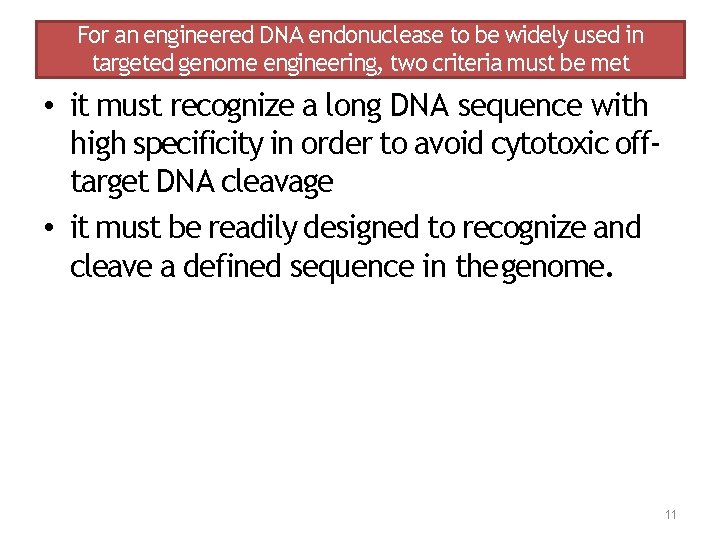
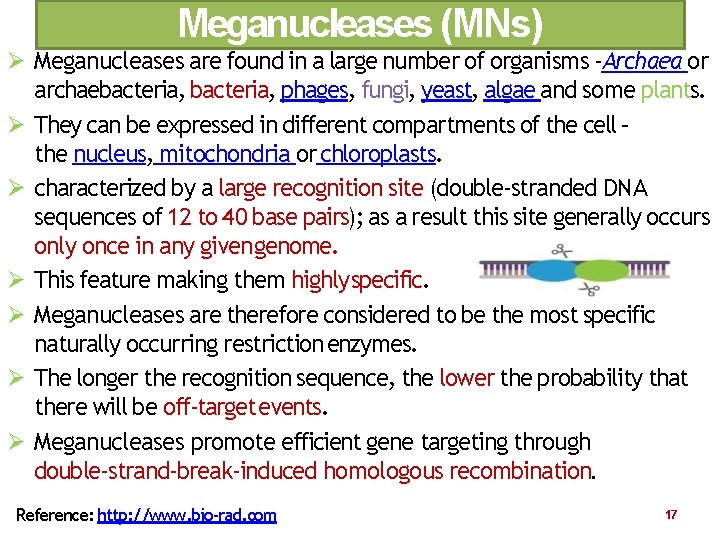
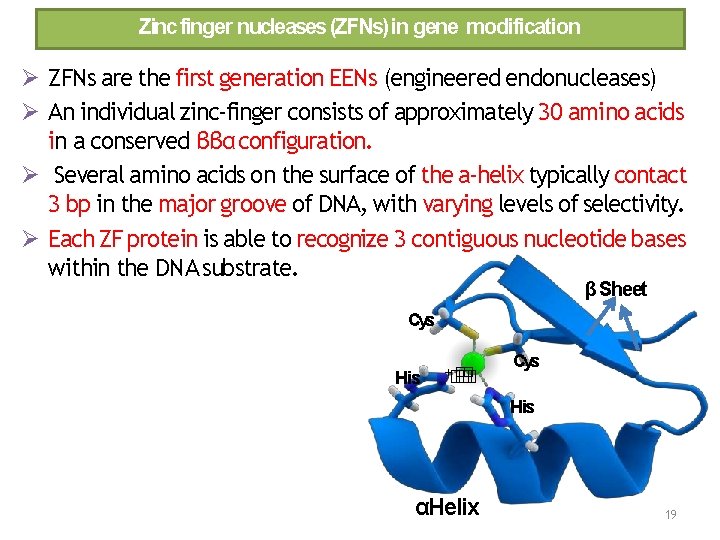
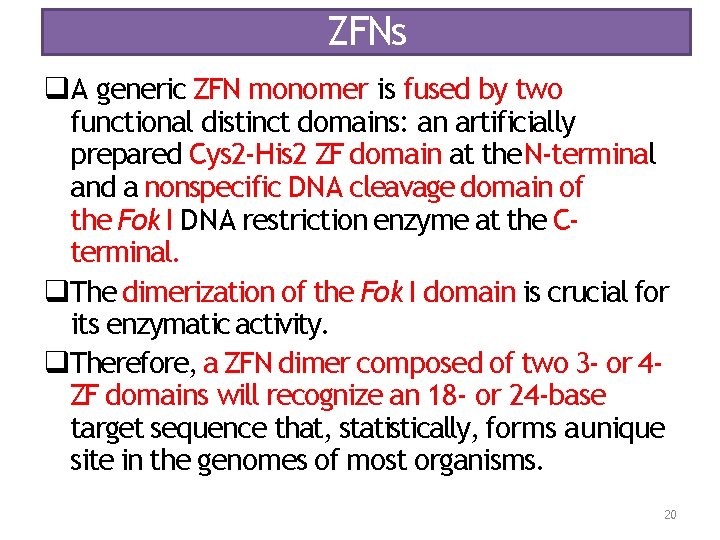
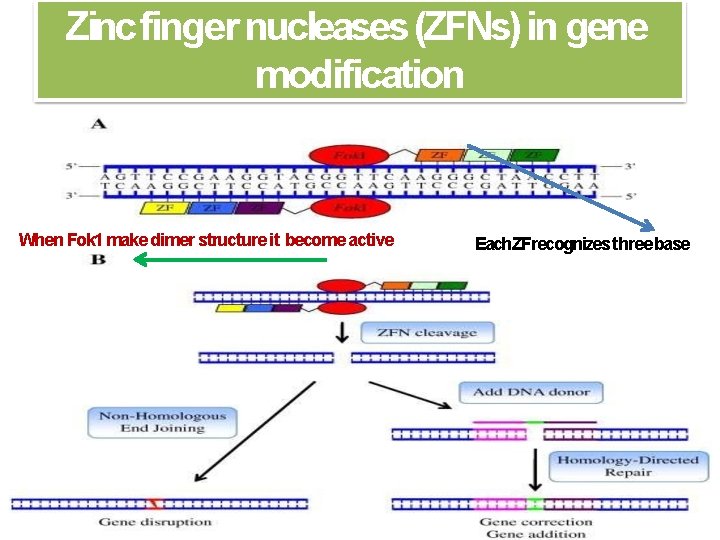
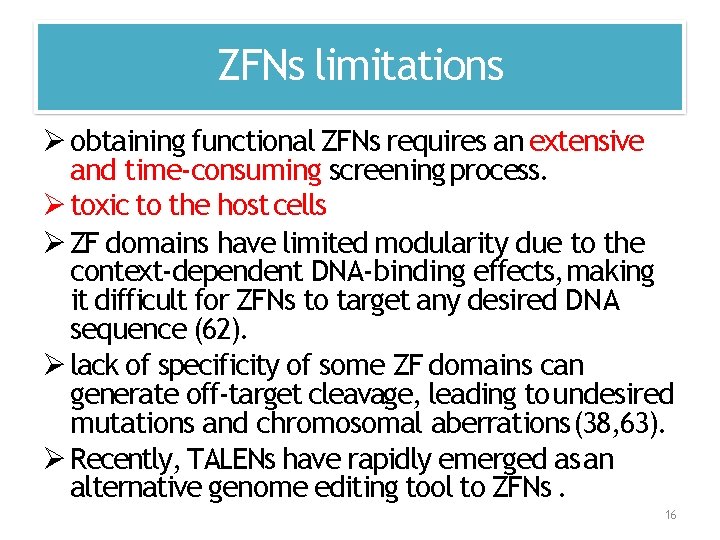
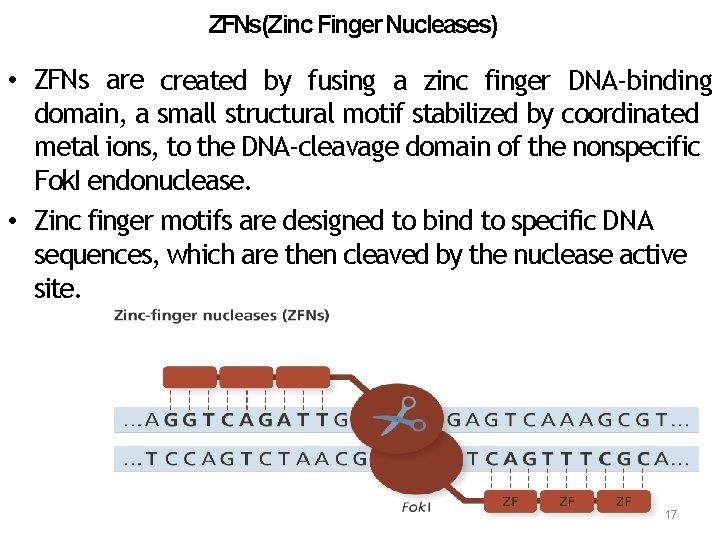
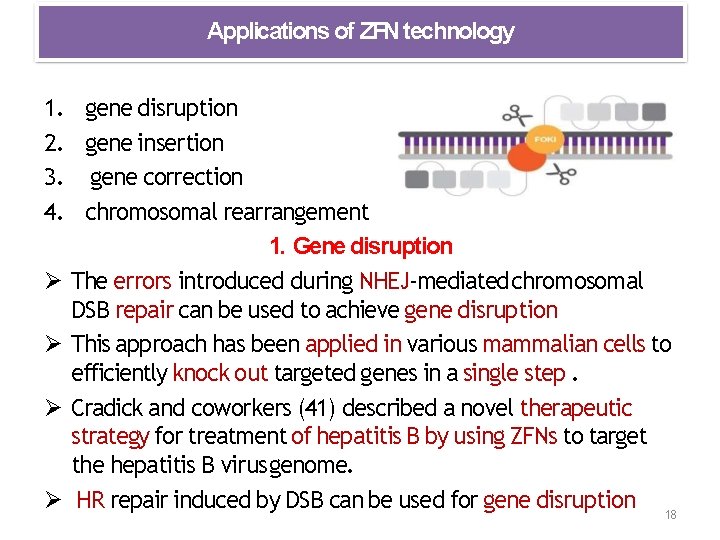
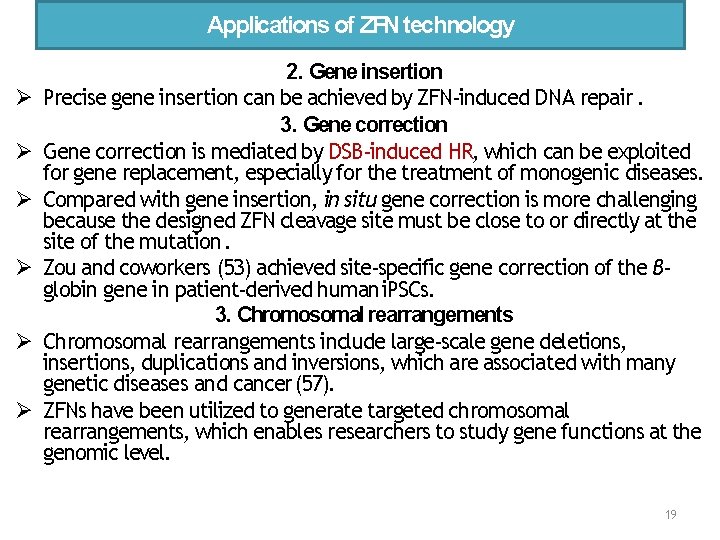
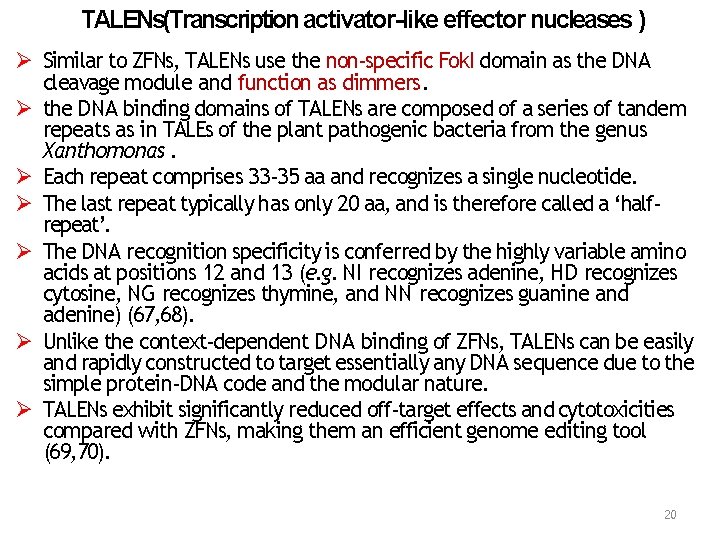
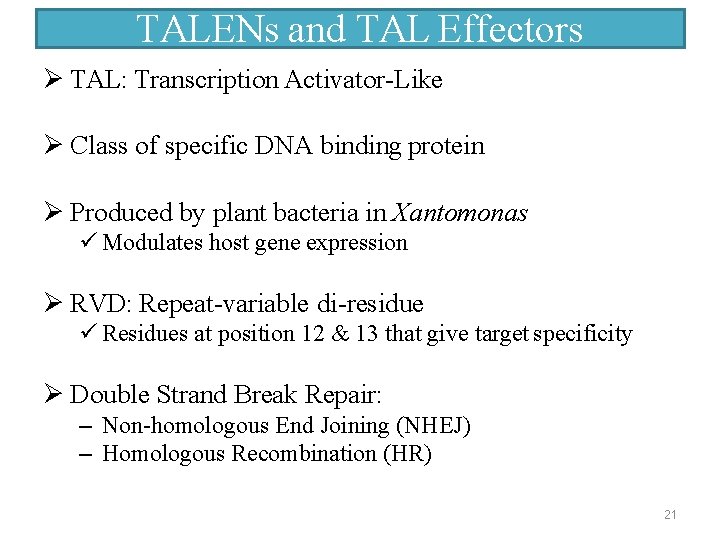
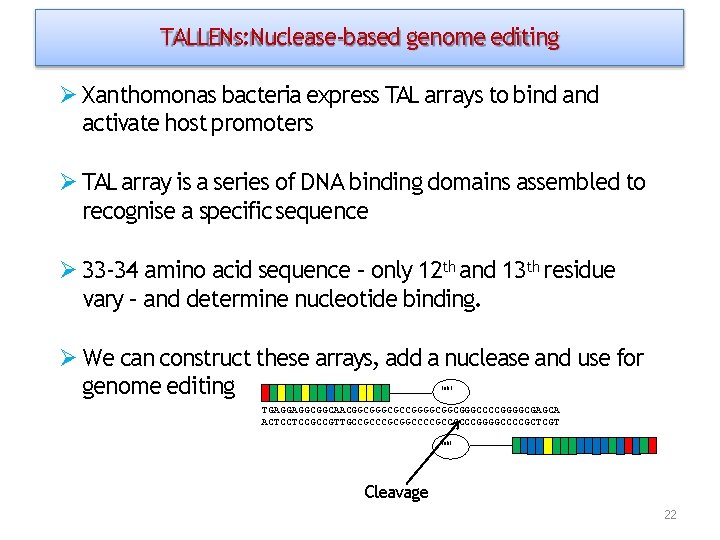
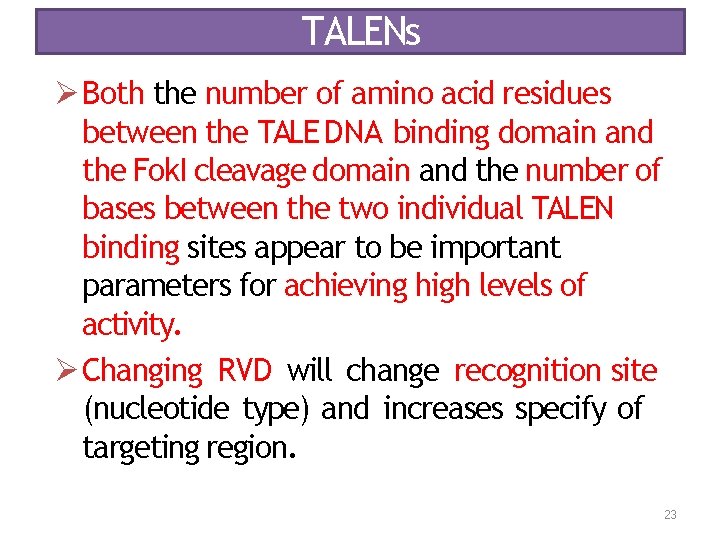
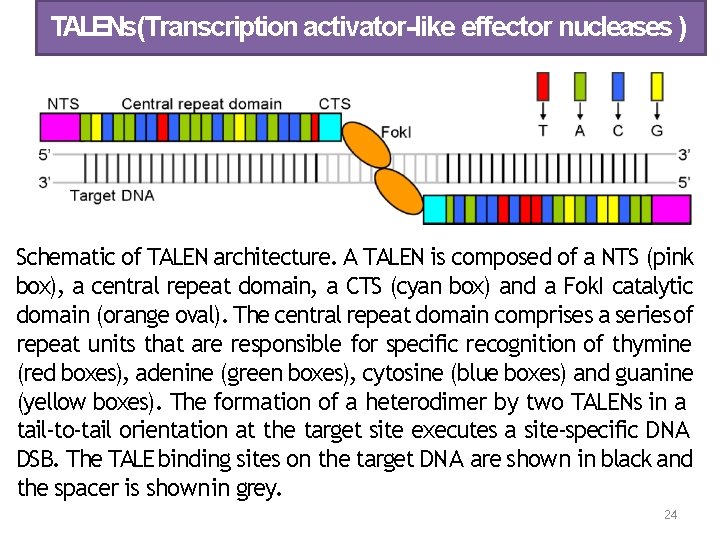
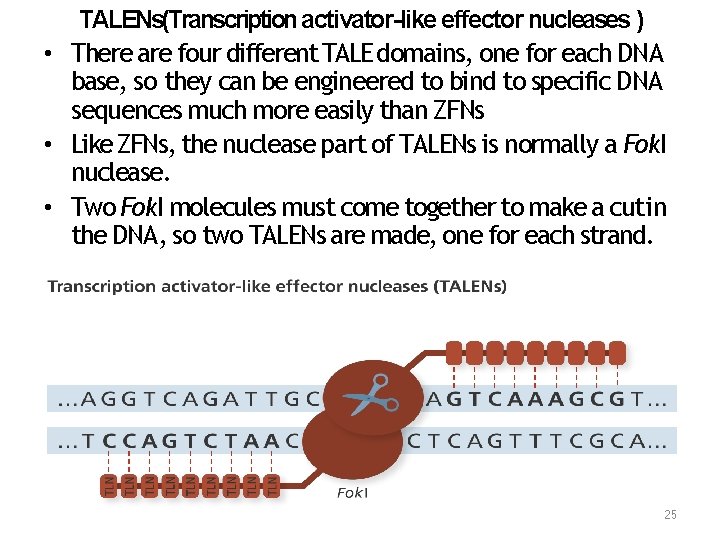
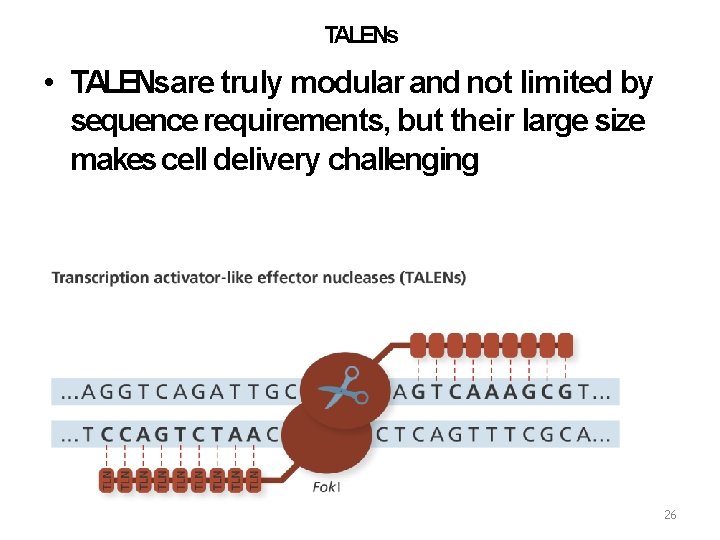
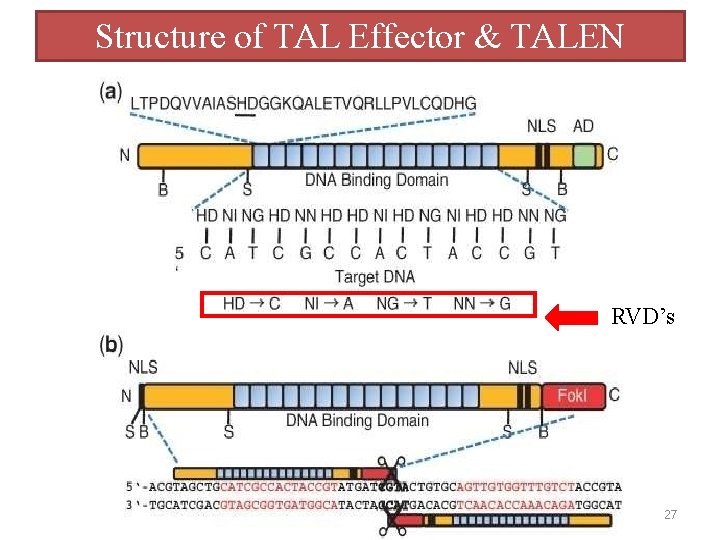
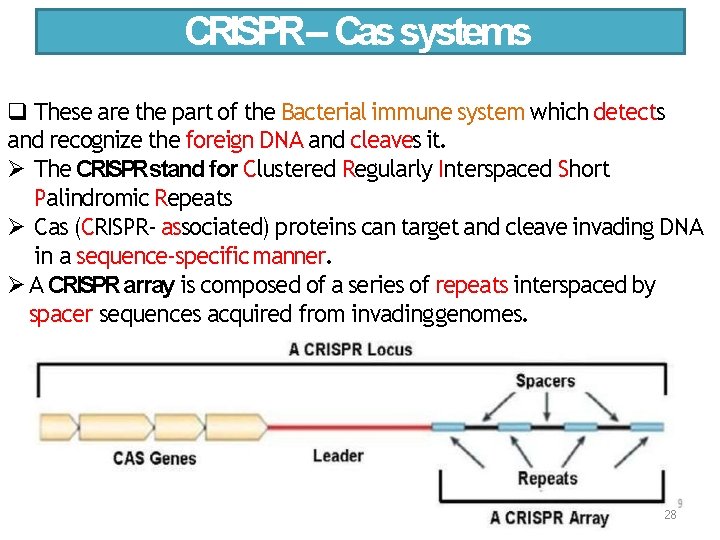
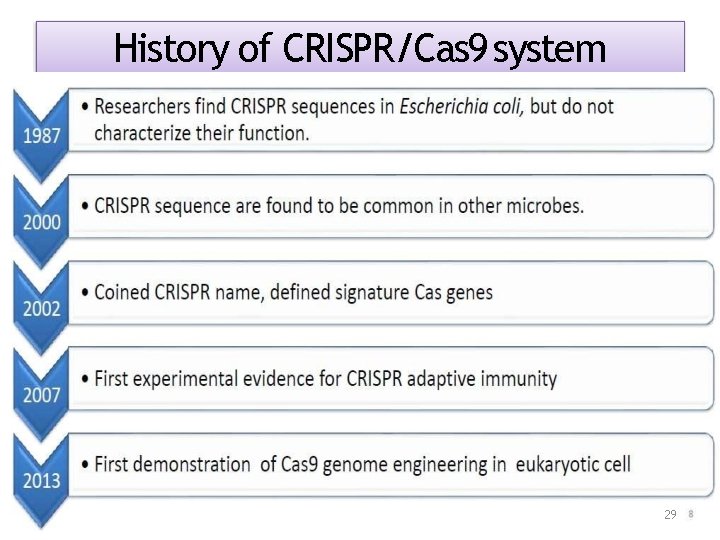
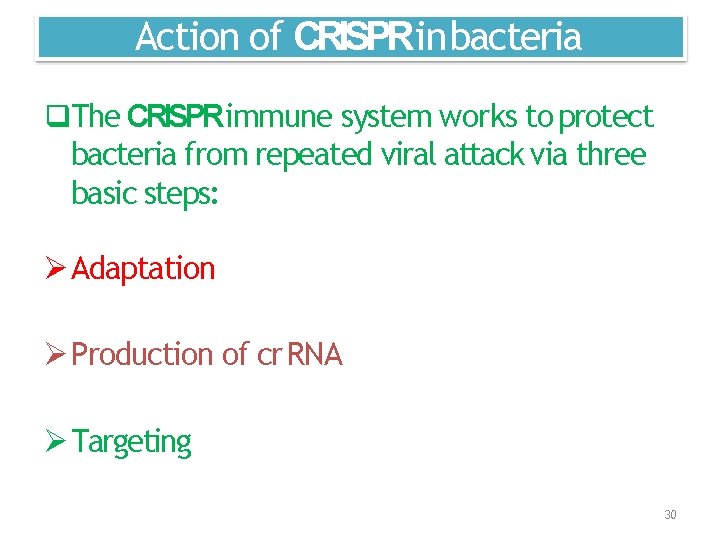
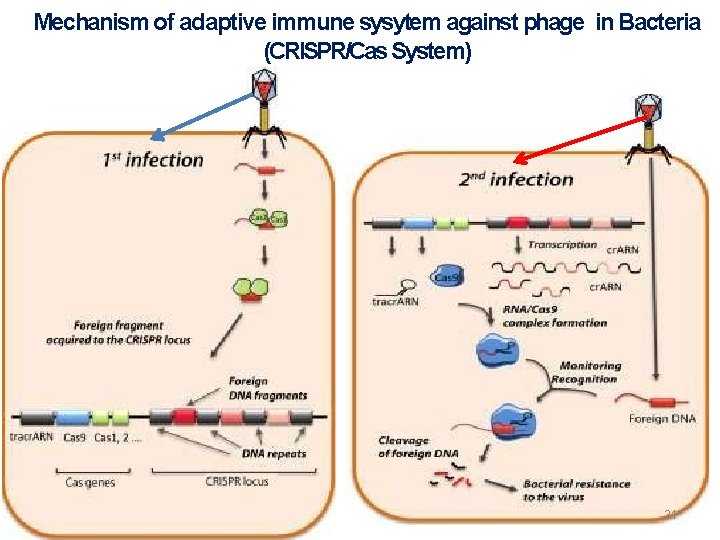
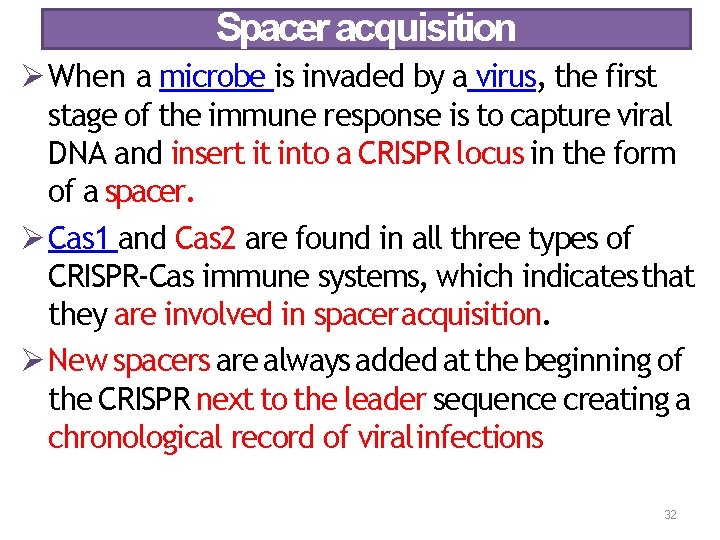
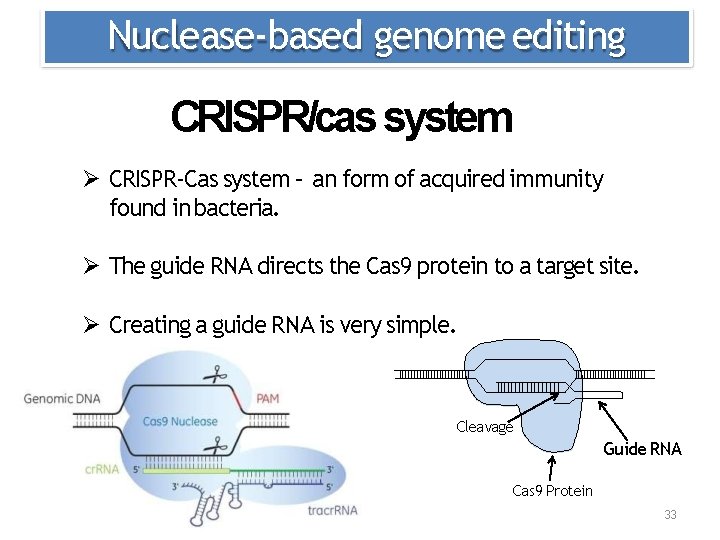
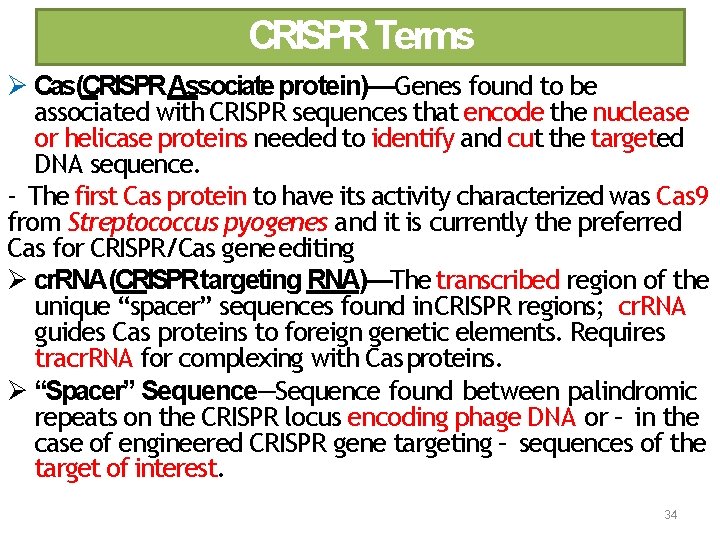
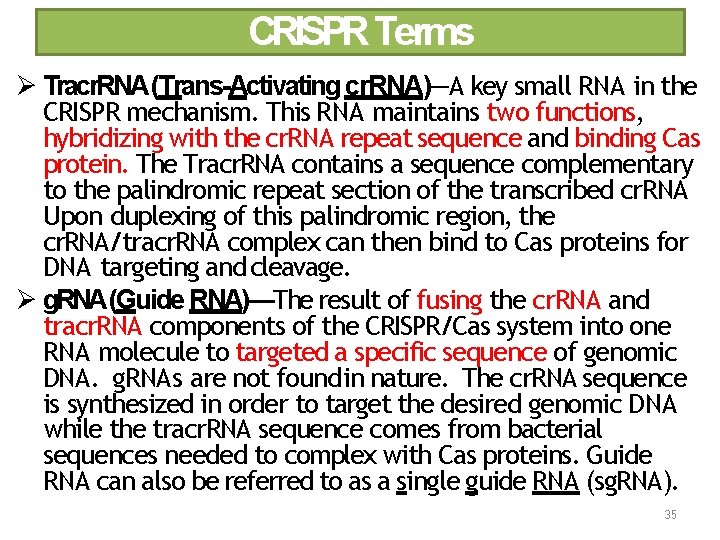
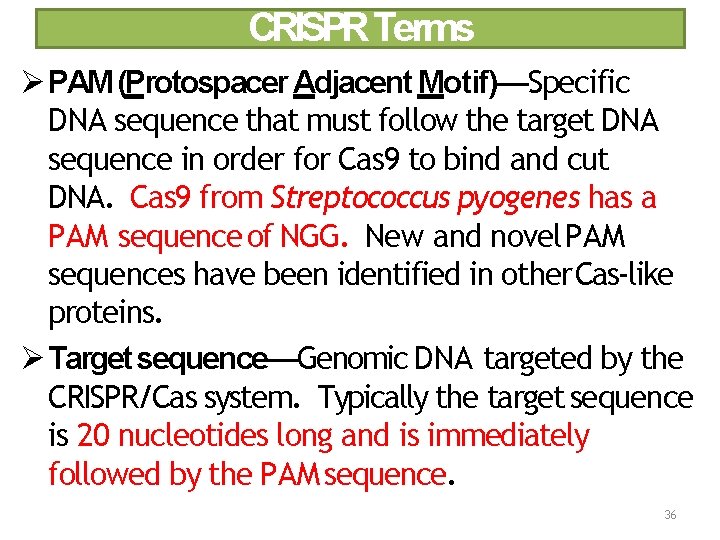
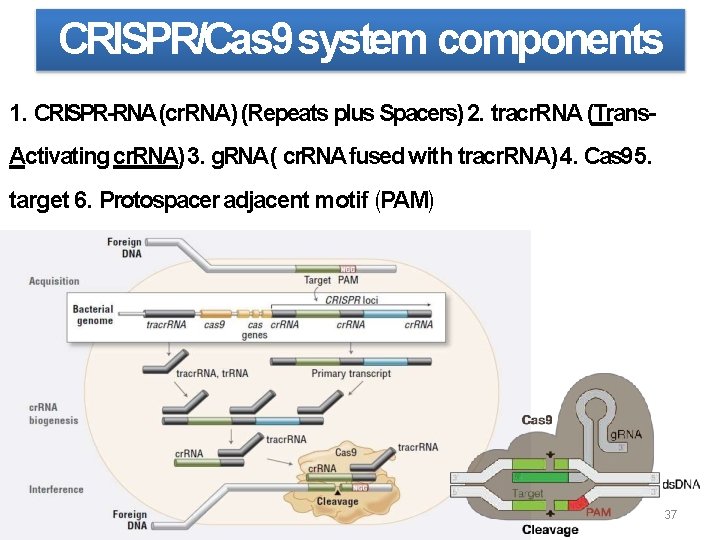
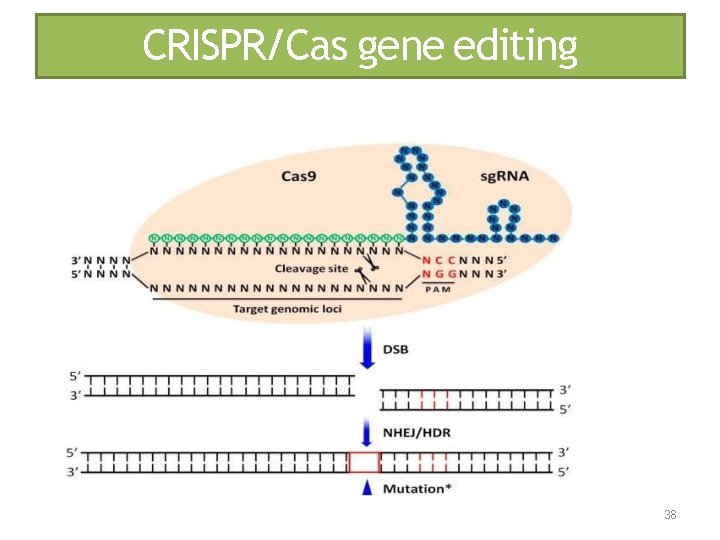
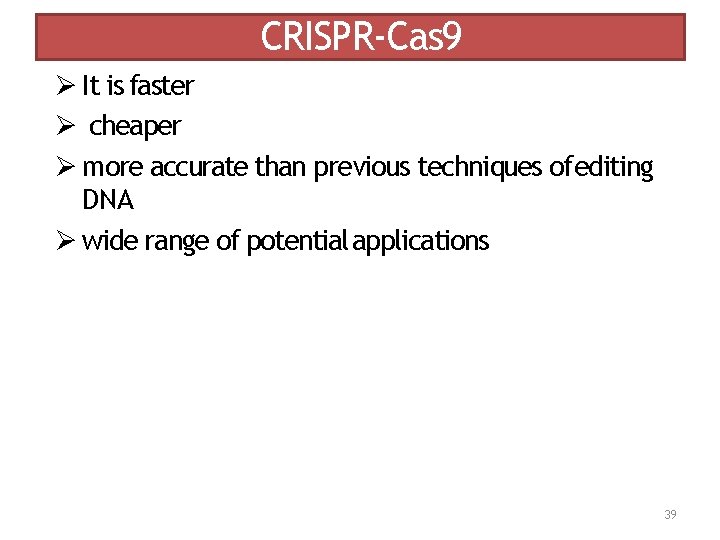
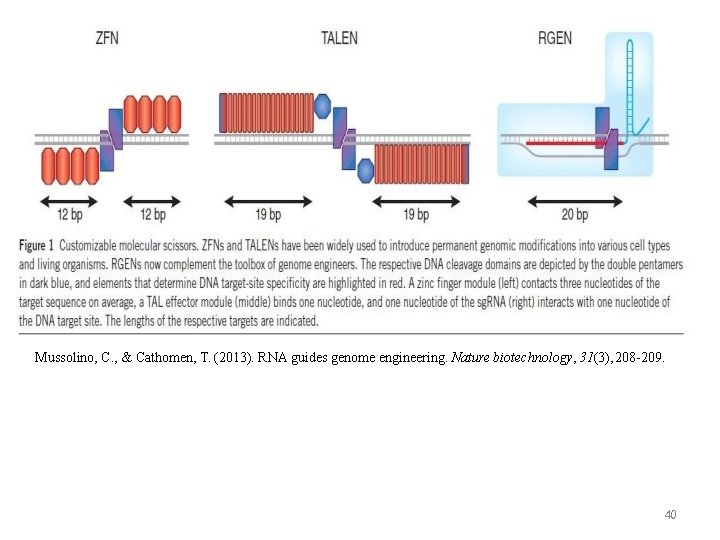
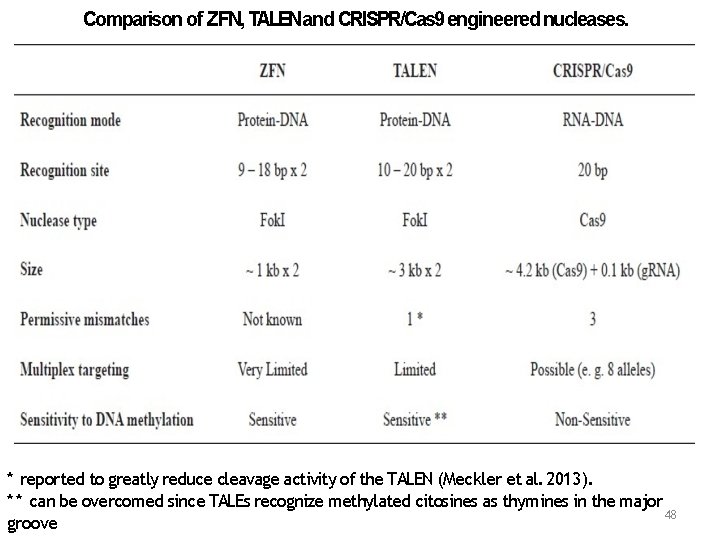
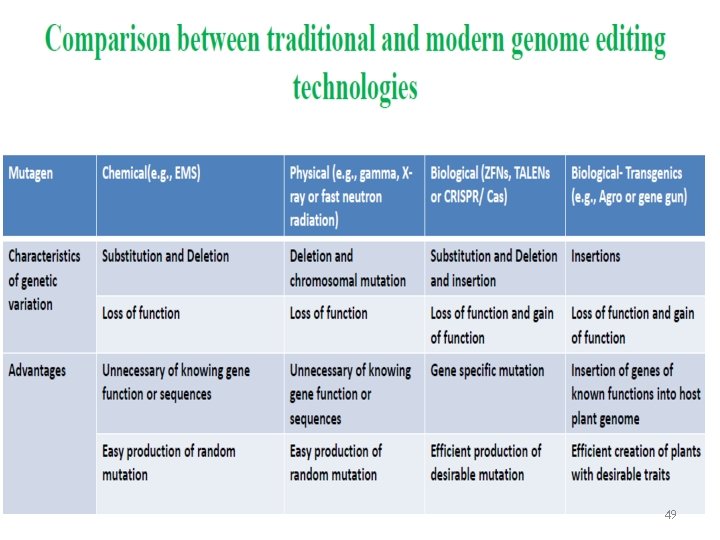
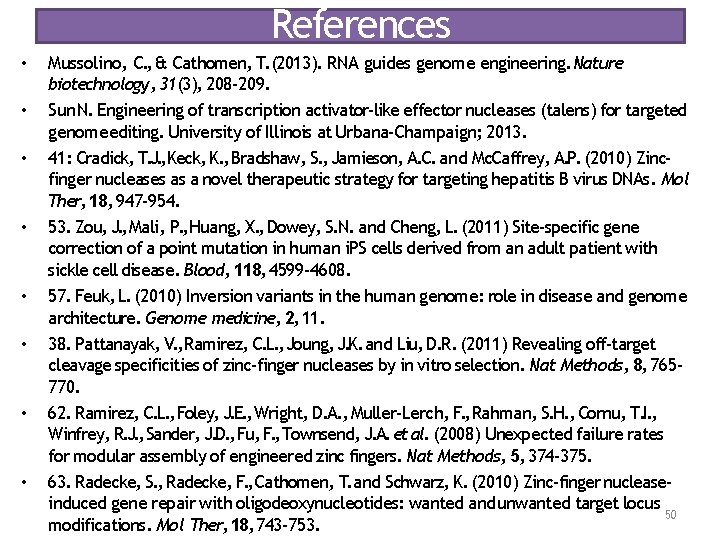
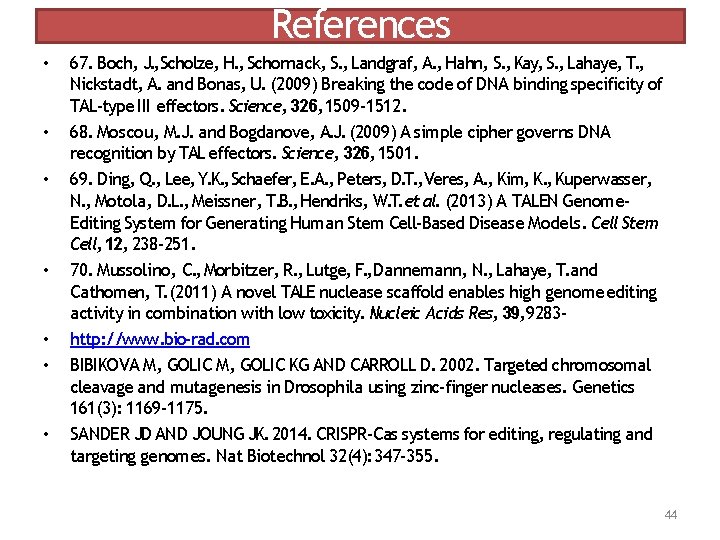
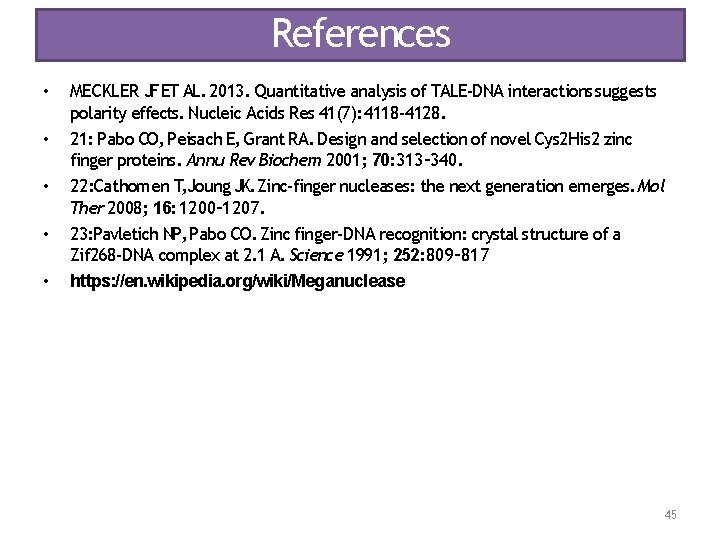
- Slides: 45
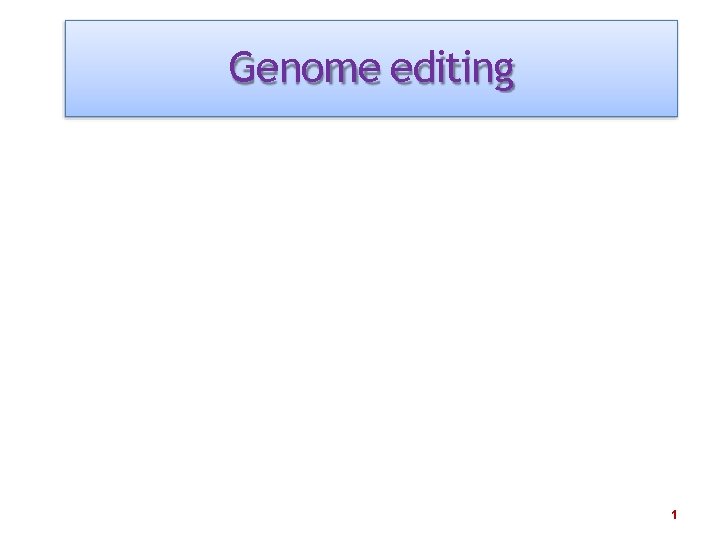
Genome editing 1
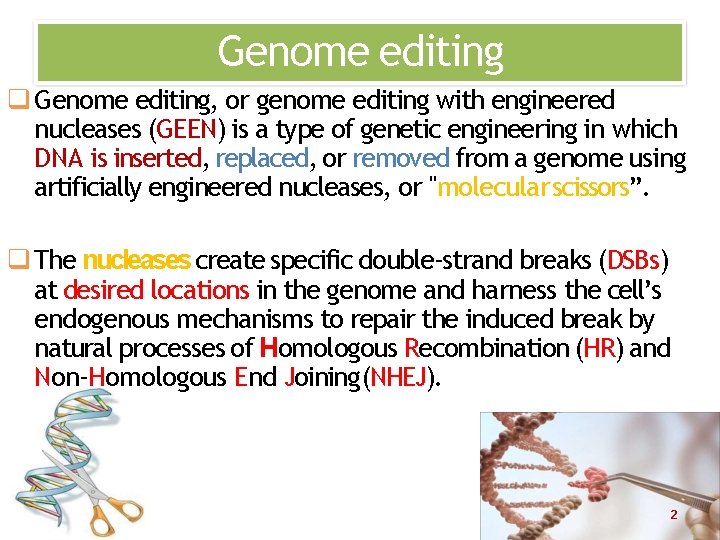
Genome editing Genome editing, or genome editing with engineered nucleases (GEEN) is a type of genetic engineering in which DNA is inserted, replaced, or removed from a genome using artificially engineered nucleases, or "molecular scissors”. The nucleases create specific double‐strand breaks (DSBs) at desired locations in the genome and harness the cell’s endogenous mechanisms to repair the induced break by natural processes of Homologous Recombination (HR) and Non‐Homologous End Joining (NHEJ). 2
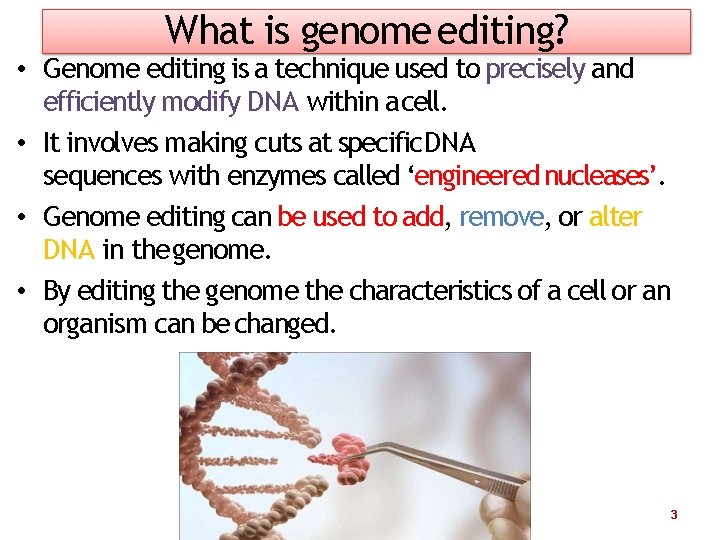
What is genome editing? • Genome editing is a technique used to precisely and efficiently modify DNA within a cell. • It involves making cuts at specific DNA sequences with enzymes called ‘engineered nucleases’. • Genome editing can be used to add, remove, or alter DNA in the genome. • By editing the genome the characteristics of a cell or an organism can be changed. 3
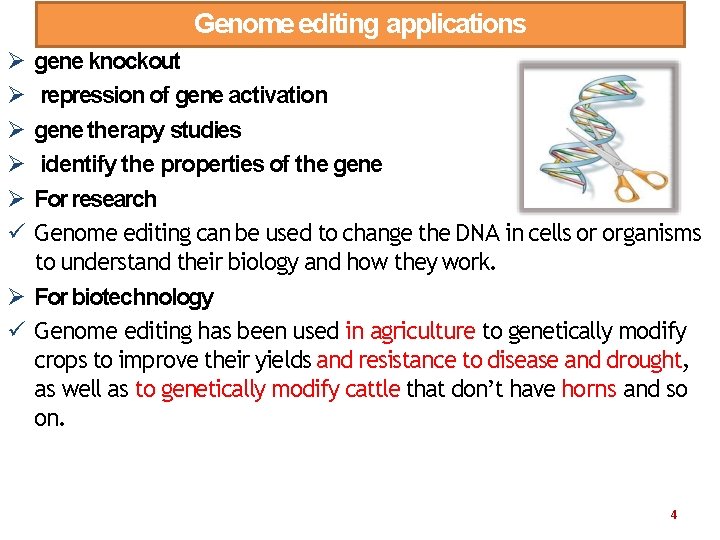
Genome editing applications gene knockout repression of gene activation gene therapy studies identify the properties of the gene For research Genome editing can be used to change the DNA in cells or organisms to understand their biology and how they work. For biotechnology Genome editing has been used in agriculture to genetically modify crops to improve their yields and resistance to disease and drought, as well as to genetically modify cattle that don’t have horns and so on. 4
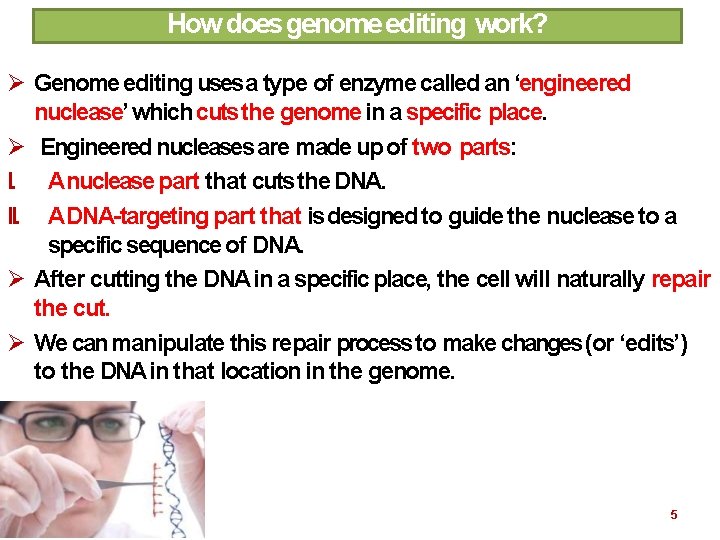
How does genome editing work? Genome editing uses a type of enzyme called an ‘engineered nuclease’ which cuts the genome in a specific place. Engineered nucleases are made up of two parts: I. A nuclease part that cuts the DNA. II. A DNA-targeting part that is designed to guide the nuclease to a specific sequence of DNA. After cutting the DNA in a specific place, the cell will naturally repair the cut. We can manipulate this repair process to make changes (or ‘edits’) to the DNA in that location in the genome. 5
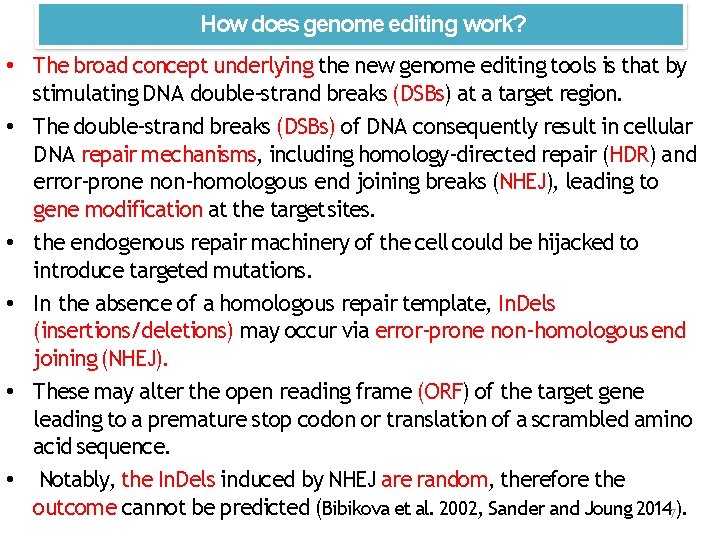
How does genome editing work? • The broad concept underlying the new genome editing tools is that by stimulating DNA double‐strand breaks (DSBs) at a target region. • The double‐strand breaks (DSBs) of DNA consequently result in cellular DNA repair mechanisms, including homology‐directed repair (HDR) and error‐prone non‐homologous end joining breaks (NHEJ), leading to gene modification at the target sites. • the endogenous repair machinery of the cell could be hijacked to introduce targeted mutations. • In the absence of a homologous repair template, In. Dels (insertions/deletions) may occur via error‐prone non‐homologous end joining (NHEJ). • These may alter the open reading frame (ORF) of the target gene leading to a premature stop codon or translation of a scrambled amino acid sequence. • Notably, the In. Dels induced by NHEJ are random, therefore the outcome cannot be predicted (Bibikova et al. 2002, Sander and Joung 20147).
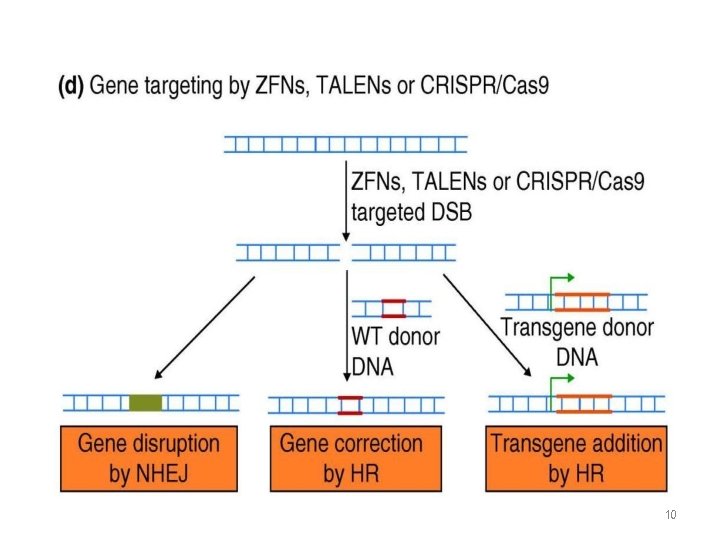
10
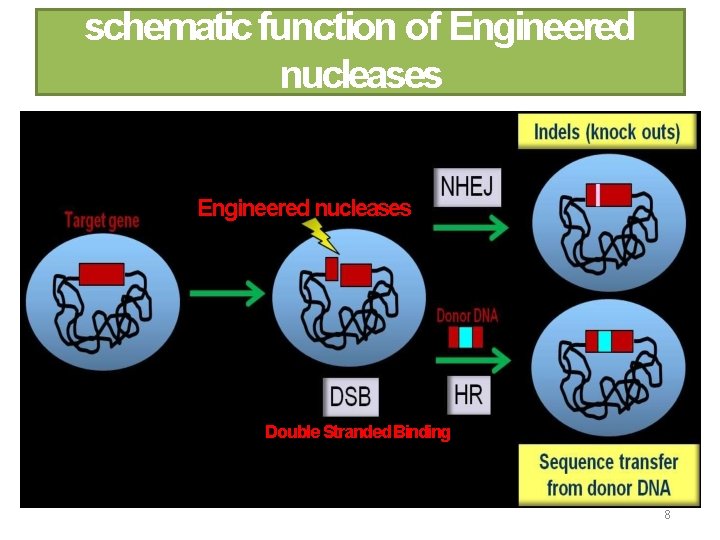
schematic function of Engineered nucleases Double Stranded Binding 8
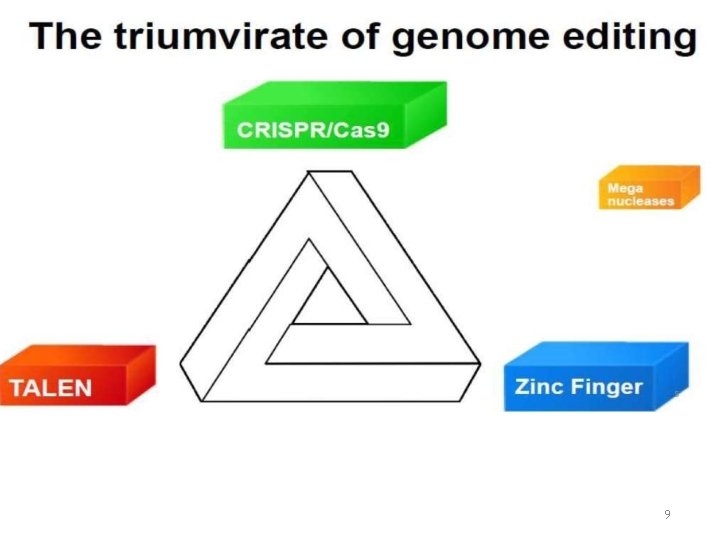
9
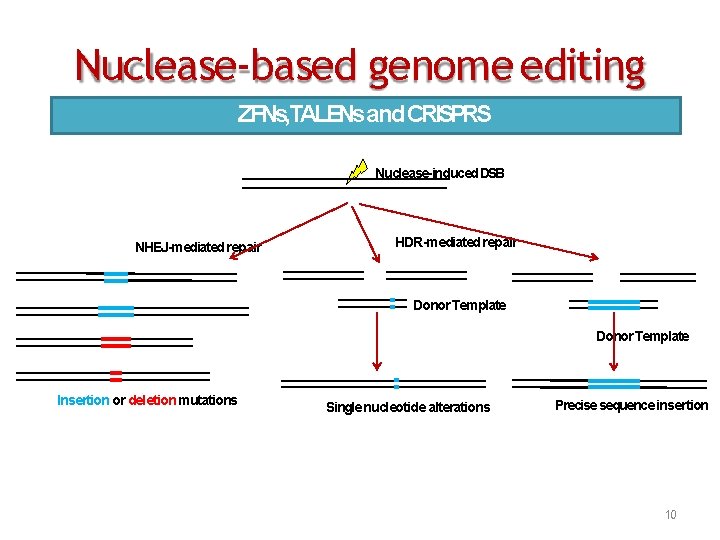
Nuclease‐based genome editing ZFNs, TALENsand CRISPRS Nuclease-induced DSB NHEJ-mediated repair HDR-mediated repair Donor Template Insertion or deletion mutations Single nucleotide alterations Precise sequence insertion 10
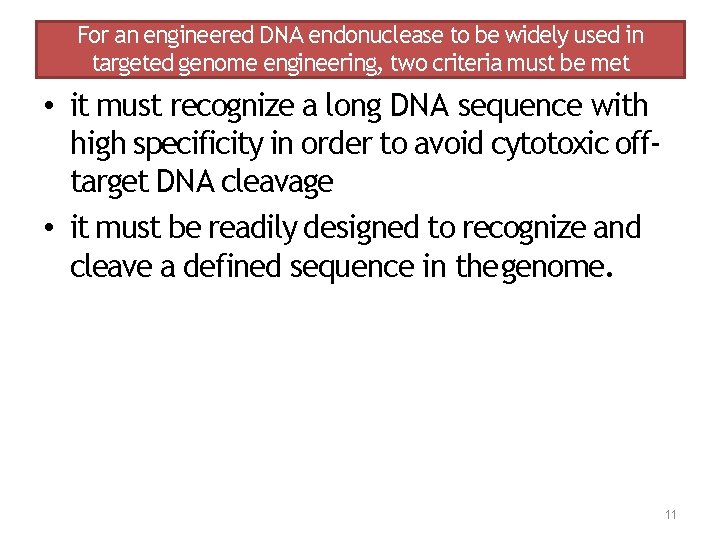
For an engineered DNA endonuclease to be widely used in targeted genome engineering, two criteria must be met • it must recognize a long DNA sequence with high specificity in order to avoid cytotoxic off‐ target DNA cleavage • it must be readily designed to recognize and cleave a defined sequence in the genome. 11
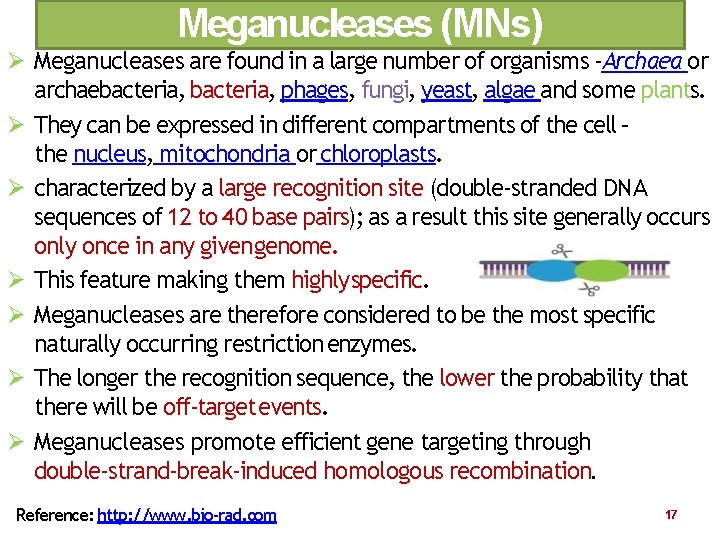
Meganucleases (MNs) Meganucleases are found in a large number of organisms ‐Archaea or archaebacteria, phages, fungi, yeast, algae and some plants. They can be expressed in different compartments of the cell – the nucleus, mitochondria or chloroplasts. characterized by a large recognition site (double‐stranded DNA sequences of 12 to 40 base pairs); as a result this site generally occurs only once in any given genome. This feature making them highly specific. Meganucleases are therefore considered to be the most specific naturally occurring restriction enzymes. The longer the recognition sequence, the lower the probability that there will be off‐target events. Meganucleases promote efficient gene targeting through double‐strand‐break‐induced homologous recombination. Reference: http: //www. bio‐rad. com 17
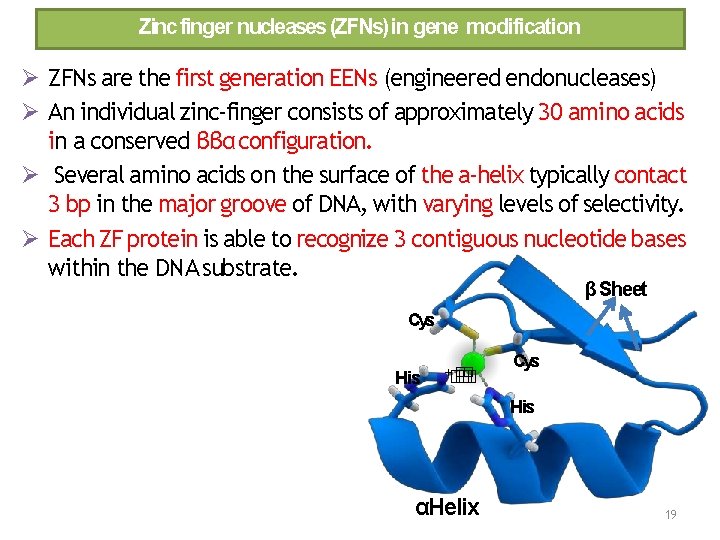
Zinc finger nucleases (ZFNs) in gene modification ZFNs are the first generation EENs (engineered endonucleases) An individual zinc‐finger consists of approximately 30 amino acids in a conserved ββα configuration. Several amino acids on the surface of the a‐helix typically contact 3 bp in the major groove of DNA, with varying levels of selectivity. Each ZF protein is able to recognize 3 contiguous nucleotide bases within the DNA substrate. β Sheet Cys His +� � � Cys His αHelix 19
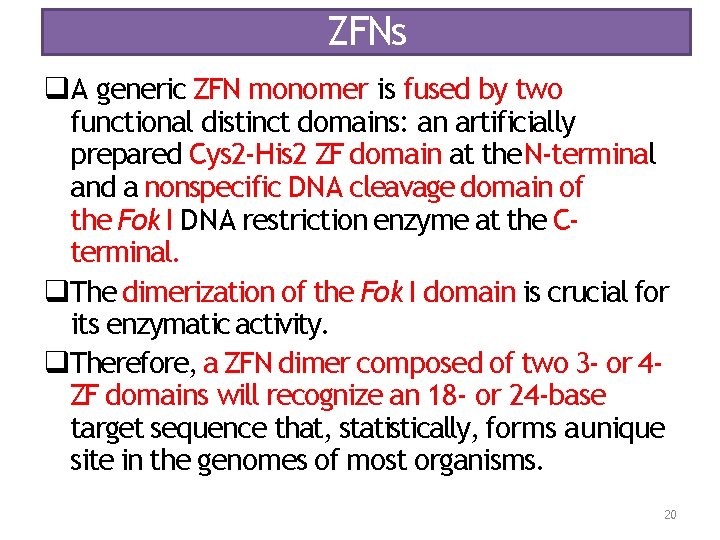
ZFNs A generic ZFN monomer is fused by two functional distinct domains: an artificially prepared Cys 2‐His 2 ZF domain at the N‐terminal and a nonspecific DNA cleavage domain of the Fok I DNA restriction enzyme at the C‐ terminal. The dimerization of the Fok I domain is crucial for its enzymatic activity. Therefore, a ZFN dimer composed of two 3‐ or 4‐ ZF domains will recognize an 18‐ or 24‐base target sequence that, statistically, forms aunique site in the genomes of most organisms. 20
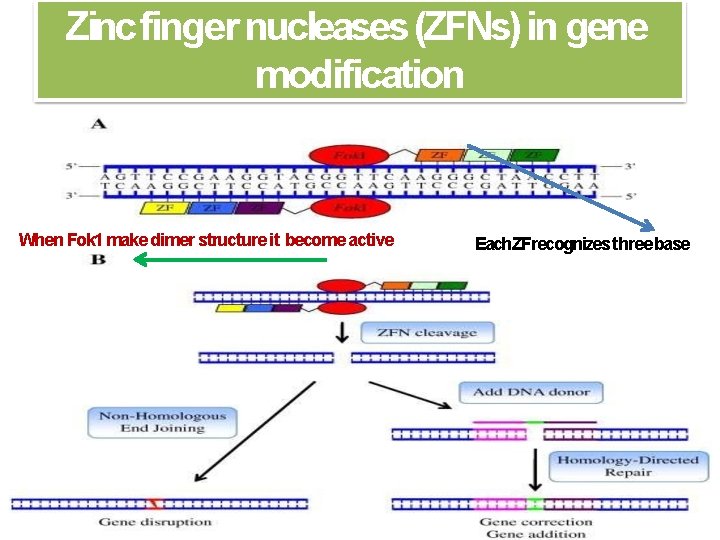
Zinc finger nucleases (ZFNs) in gene modification When Fok 1 make dimer structure it become active Each ZFrecognizes three base 21
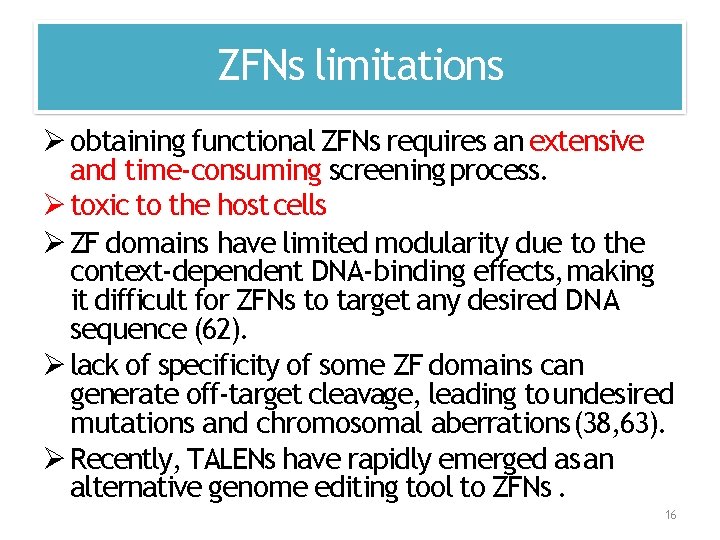
ZFNs limitations obtaining functional ZFNs requires an extensive and time‐consuming screening process. toxic to the host cells ZF domains have limited modularity due to the context‐dependent DNA‐binding effects, making it difficult for ZFNs to target any desired DNA sequence (62). lack of specificity of some ZF domains can generate off‐target cleavage, leading to undesired mutations and chromosomal aberrations (38, 63). Recently, TALENs have rapidly emerged as an alternative genome editing tool to ZFNs. 16
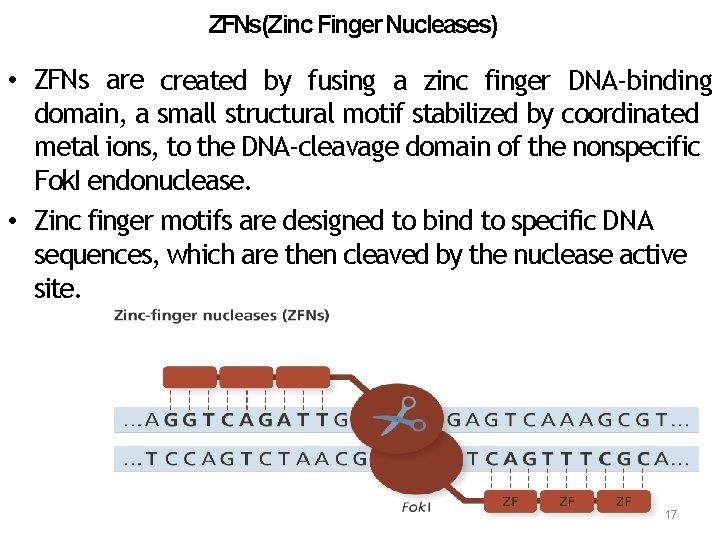
ZFNs(Zinc Finger Nucleases) • ZFNs are created by fusing a zinc finger DNA‐binding domain, a small structural motif stabilized by coordinated metal ions, to the DNA‐cleavage domain of the nonspecific Fok. I endonuclease. • Zinc finger motifs are designed to bind to specific DNA sequences, which are then cleaved by the nuclease active site. 17
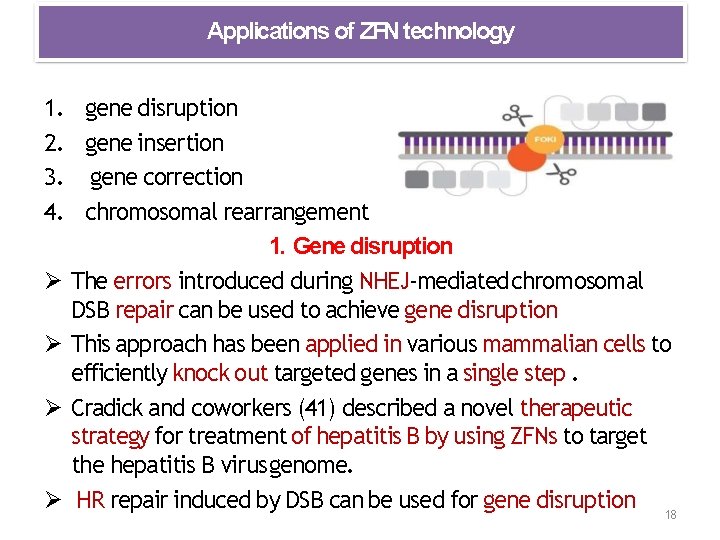
Applications of ZFN technology 1. gene disruption 2. gene insertion 3. gene correction 4. chromosomal rearrangement 1. Gene disruption The errors introduced during NHEJ‐mediated chromosomal DSB repair can be used to achieve gene disruption This approach has been applied in various mammalian cells to efficiently knock out targeted genes in a single step. Cradick and coworkers (41) described a novel therapeutic strategy for treatment of hepatitis B by using ZFNs to target the hepatitis B virus genome. HR repair induced by DSB can be used for gene disruption 18
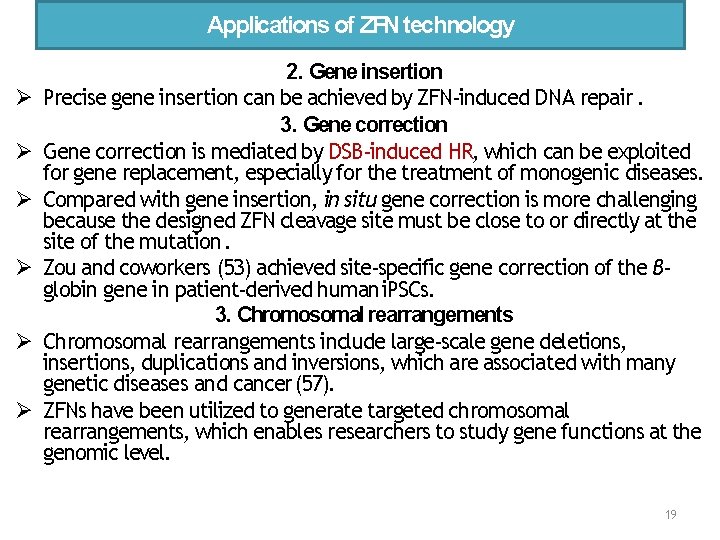
Applications of ZFN technology 2. Gene insertion Precise gene insertion can be achieved by ZFN‐induced DNA repair. 3. Gene correction is mediated by DSB‐induced HR, which can be exploited for gene replacement, especially for the treatment of monogenic diseases. Compared with gene insertion, in situ gene correction is more challenging because the designed ZFN cleavage site must be close to or directly at the site of the mutation. Zou and coworkers (53) achieved site‐specific gene correction of the β‐ globin gene in patient‐derived human i. PSCs. 3. Chromosomal rearrangements include large‐scale gene deletions, insertions, duplications and inversions, which are associated with many genetic diseases and cancer (57). ZFNs have been utilized to generate targeted chromosomal rearrangements, which enables researchers to study gene functions at the genomic level. 19
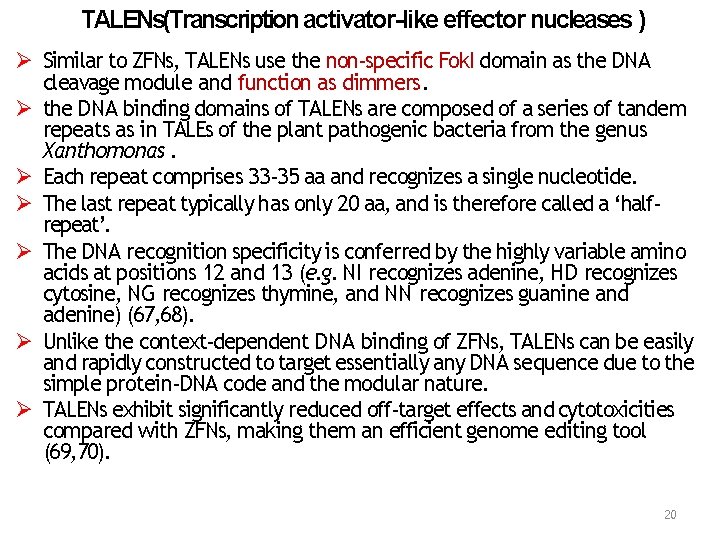
TALENs(Transcription activator-like effector nucleases ) Similar to ZFNs, TALENs use the non‐specific Fok. I domain as the DNA cleavage module and function as dimmers. the DNA binding domains of TALENs are composed of a series of tandem repeats as in TALEs of the plant pathogenic bacteria from the genus Xanthomonas. Each repeat comprises 33‐ 35 aa and recognizes a single nucleotide. The last repeat typically has only 20 aa, and is therefore called a ‘half‐ repeat’. The DNA recognition specificity is conferred by the highly variable amino acids at positions 12 and 13 (e. g. NI recognizes adenine, HD recognizes cytosine, NG recognizes thymine, and NN recognizes guanine and adenine) (67, 68). Unlike the context‐dependent DNA binding of ZFNs, TALENs can be easily and rapidly constructed to target essentially any DNA sequence due to the simple protein‐DNA code and the modular nature. TALENs exhibit significantly reduced off‐target effects and cytotoxicities compared with ZFNs, making them an efficient genome editing tool (69, 70). 20
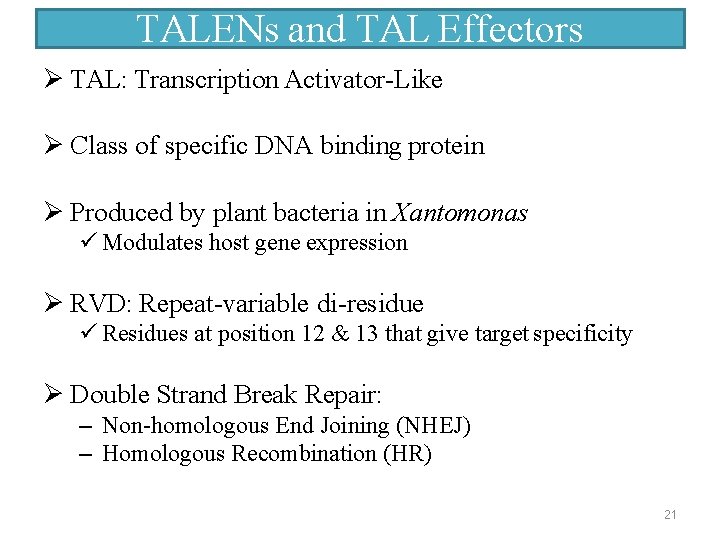
TALENs and TAL Effectors TAL: Transcription Activator-Like Class of specific DNA binding protein Produced by plant bacteria in Xantomonas Modulates host gene expression RVD: Repeat-variable di-residue Residues at position 12 & 13 that give target specificity Double Strand Break Repair: – Non-homologous End Joining (NHEJ) – Homologous Recombination (HR) 21
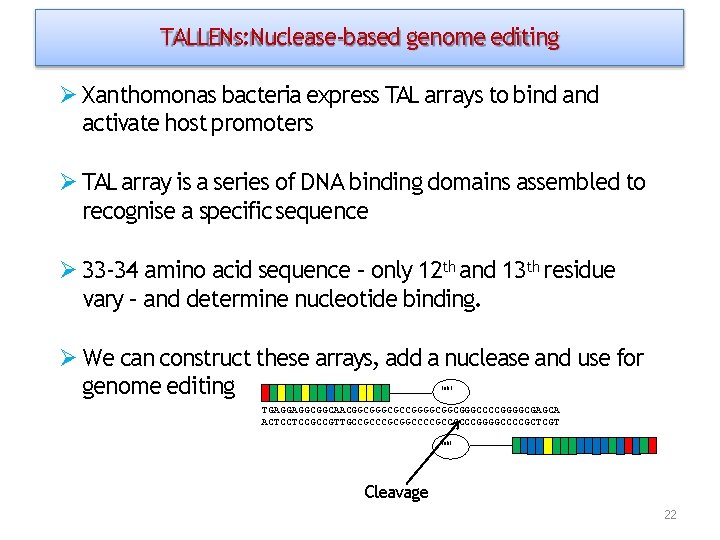
TALLENs: Nuclease‐based genome editing Xanthomonas bacteria express TAL arrays to bind activate host promoters TAL array is a series of DNA binding domains assembled to recognise a specific sequence 33‐ 34 amino acid sequence – only 12 th and 13 th residue vary – and determine nucleotide binding. We can construct these arrays, add a nuclease and use for genome editing Fok 1 TGAGGAGGCGGCAACGGCGCCGGGGCGGCGGGCCCCGGGGCGAGCA ACTCCTCCGCCGTTGCCGCGGCCCCGCCGCCCGGGGCCCCGCTCGT Fok 1 Cleavage 22
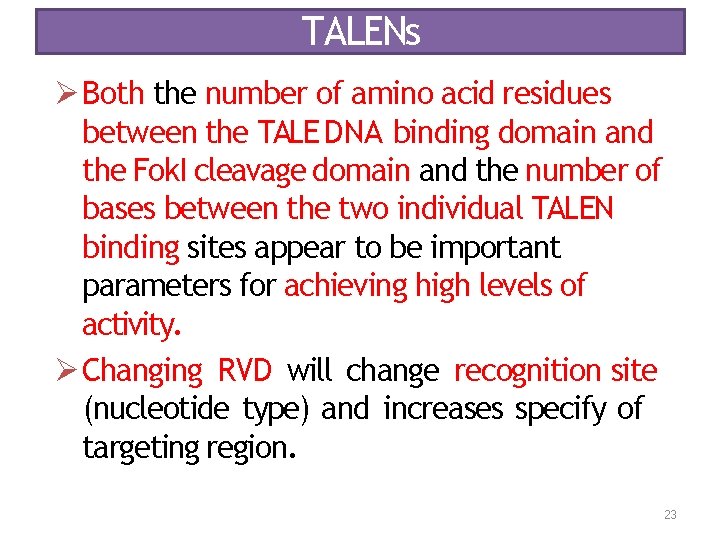
TALENs Both the number of amino acid residues between the TALE DNA binding domain and the Fok. I cleavage domain and the number of bases between the two individual TALEN binding sites appear to be important parameters for achieving high levels of activity. Changing RVD will change recognition site (nucleotide type) and increases specify of targeting region. 23
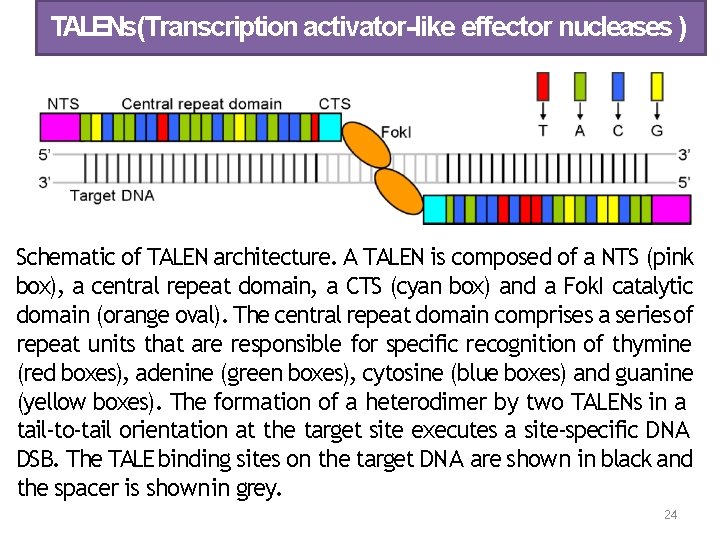
TALENs(Transcription activator-like effector nucleases ) Schematic of TALEN architecture. A TALEN is composed of a NTS (pink box), a central repeat domain, a CTS (cyan box) and a Fok. I catalytic domain (orange oval). The central repeat domain comprises a series of repeat units that are responsible for specific recognition of thymine (red boxes), adenine (green boxes), cytosine (blue boxes) and guanine (yellow boxes). The formation of a heterodimer by two TALENs in a tail‐to‐tail orientation at the target site executes a site‐specific DNA DSB. The TALE binding sites on the target DNA are shown in black and the spacer is shown in grey. 24
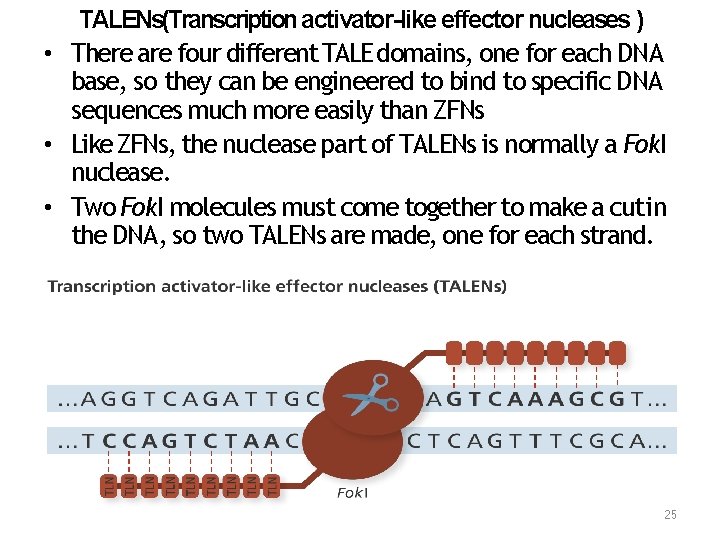
TALENs(Transcription activator-like effector nucleases ) • There are four different TALE domains, one for each DNA base, so they can be engineered to bind to specific DNA sequences much more easily than ZFNs • Like ZFNs, the nuclease part of TALENs is normally a Fok. I nuclease. • Two Fok. I molecules must come together to make a cut in the DNA, so two TALENs are made, one for each strand. 25
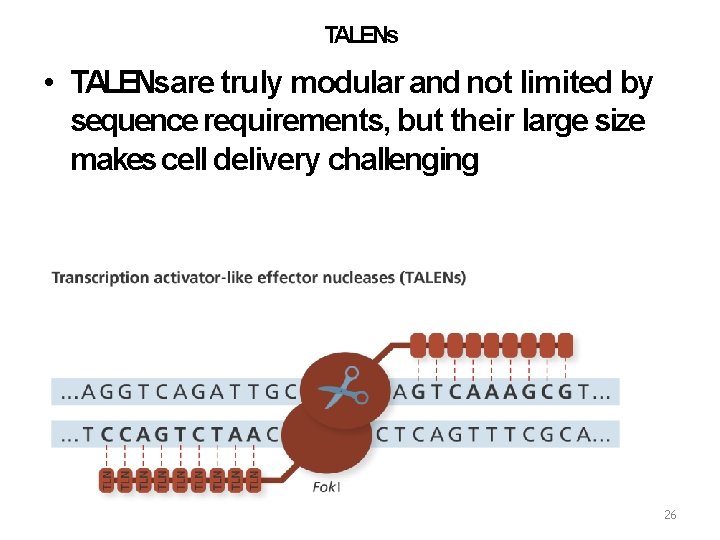
TALENs • TALENsare truly modular and not limited by sequence requirements, but their large size makes cell delivery challenging 26
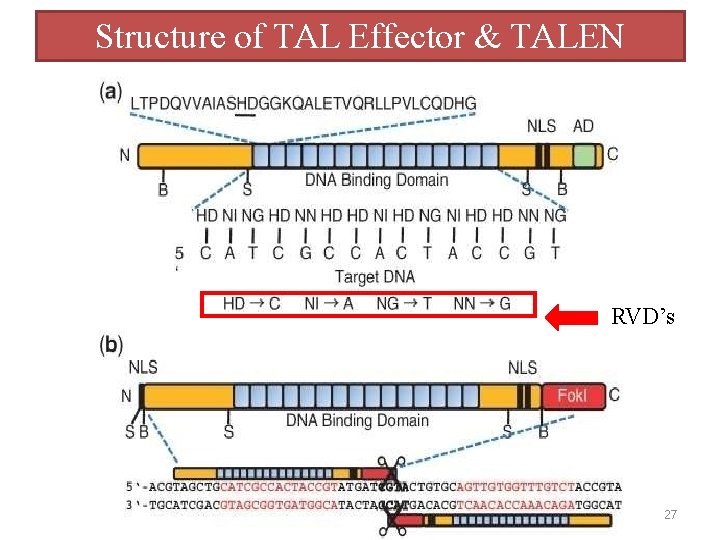
Structure of TAL Effector & TALEN RVD’s 27
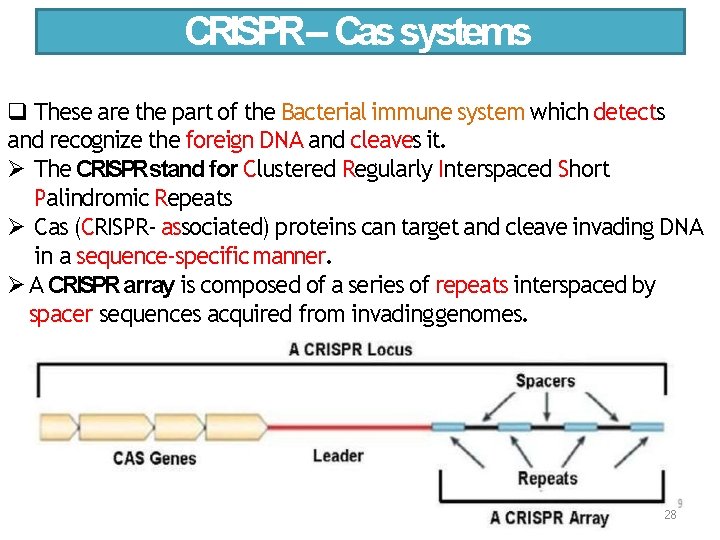
CRISPR– Cas systems These are the part of the Bacterial immune system which detects and recognize the foreign DNA and cleaves it. The CRISPRstand for Clustered Regularly Interspaced Short Palindromic Repeats Cas (CRISPR‐ associated) proteins can target and cleave invading DNA in a sequence‐specific manner. A CRISPR array is composed of a series of repeats interspaced by spacer sequences acquired from invading genomes. 28
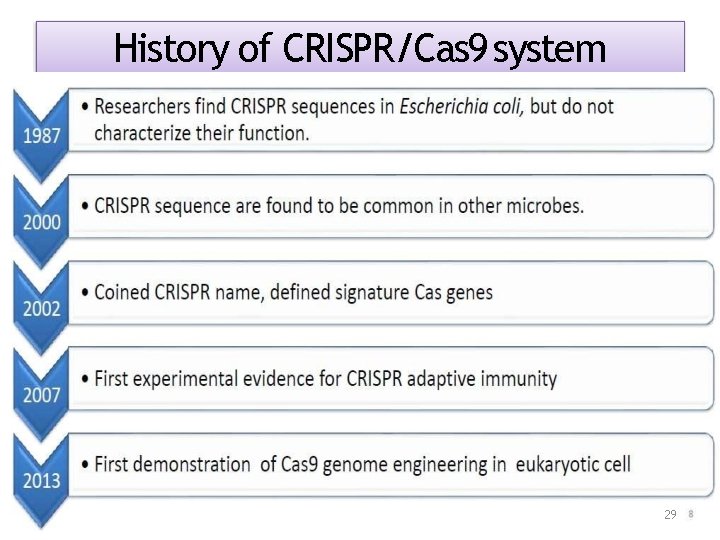
History of CRISPR/Cas 9 system 29
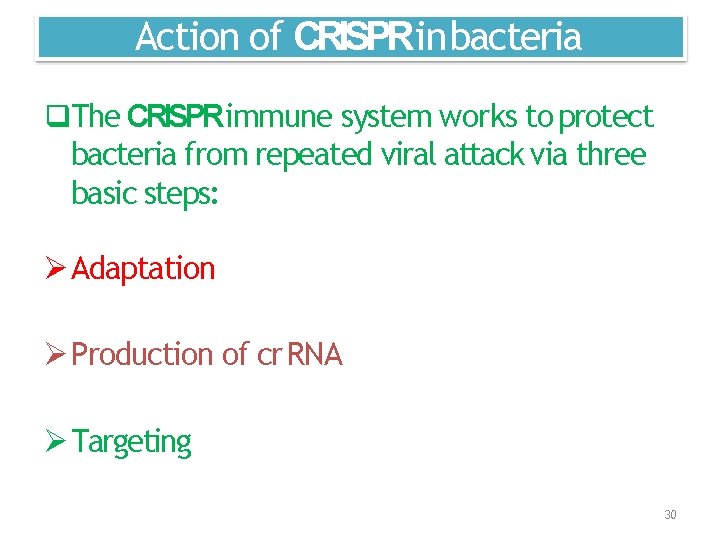
Action of CRISPRin bacteria The CRISPRimmune system works to protect bacteria from repeated viral attack via three basic steps: Adaptation Production of cr RNA Targeting 30
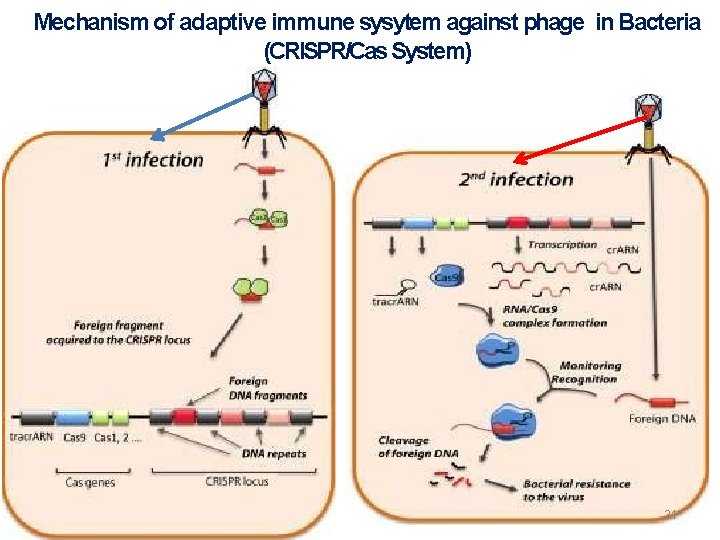
Mechanism of adaptive immune sysytem against phage in Bacteria (CRISPR/Cas System) 31
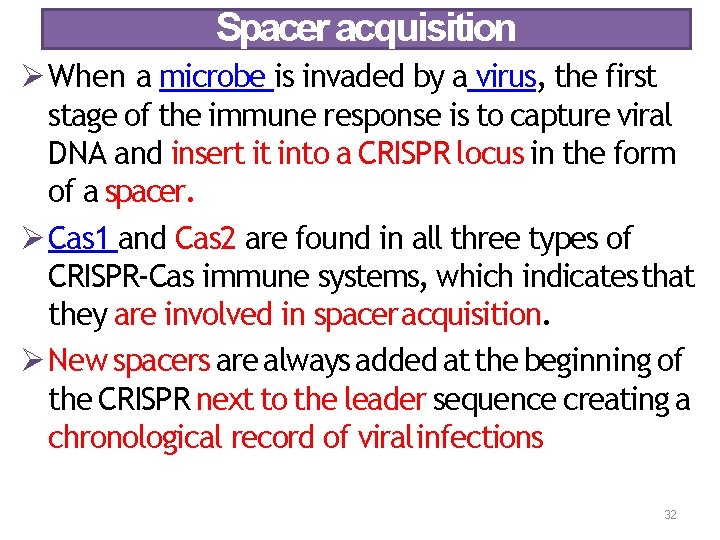
Spacer acquisition When a microbe is invaded by a virus, the first stage of the immune response is to capture viral DNA and insert it into a CRISPR locus in the form of a spacer. Cas 1 and Cas 2 are found in all three types of CRISPR‐Cas immune systems, which indicates that they are involved in spacer acquisition. New spacers are always added at the beginning of the CRISPR next to the leader sequence creating a chronological record of viral infections 32
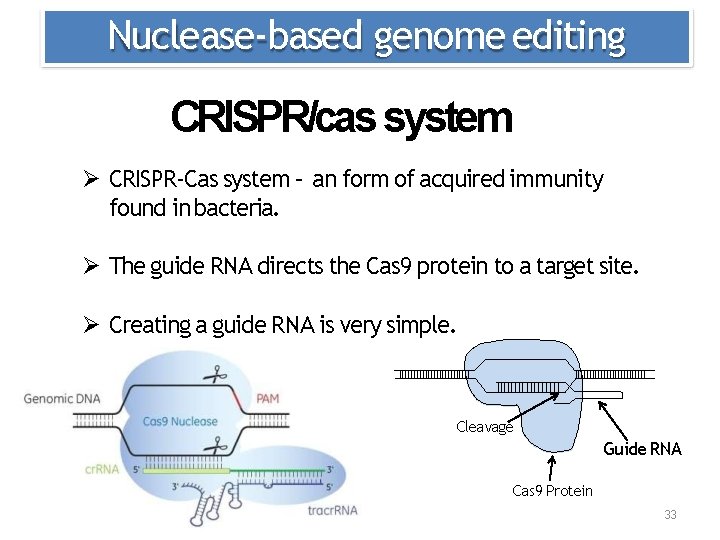
Nuclease‐based genome editing CRISPR/cas system CRISPR‐Cas system – an form of acquired immunity found in bacteria. The guide RNA directs the Cas 9 protein to a target site. Creating a guide RNA is very simple. Cleavage Guide RNA Cas 9 Protein 33
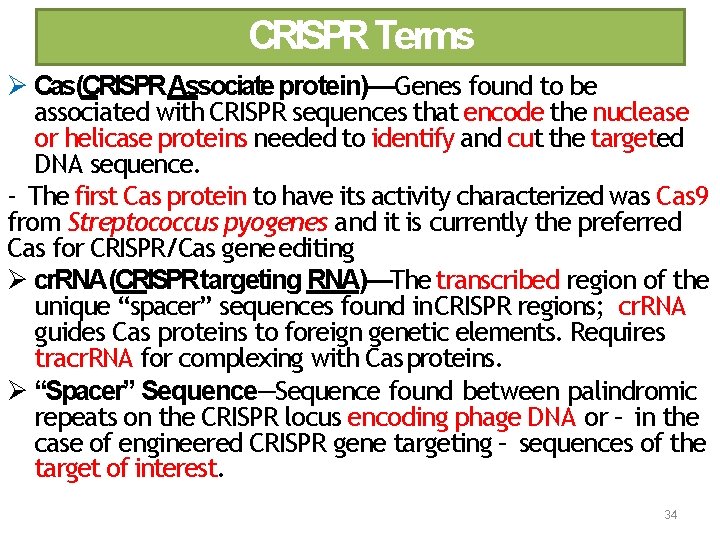
CRISPR Terms Cas(CRISPRAssociate protein)—Genes found to be associated with CRISPR sequences that encode the nuclease or helicase proteins needed to identify and cut the targeted DNA sequence. ‐ The first Cas protein to have its activity characterized was Cas 9 from Streptococcus pyogenes and it is currently the preferred Cas for CRISPR/Cas gene editing cr. RNA (CRISPRtargeting RNA)—The transcribed region of the unique “spacer” sequences found in CRISPR regions; cr. RNA guides Cas proteins to foreign genetic elements. Requires tracr. RNA for complexing with Cas proteins. “Spacer” Sequence—Sequence found between palindromic repeats on the CRISPR locus encoding phage DNA or – in the case of engineered CRISPR gene targeting – sequences of the target of interest. 34
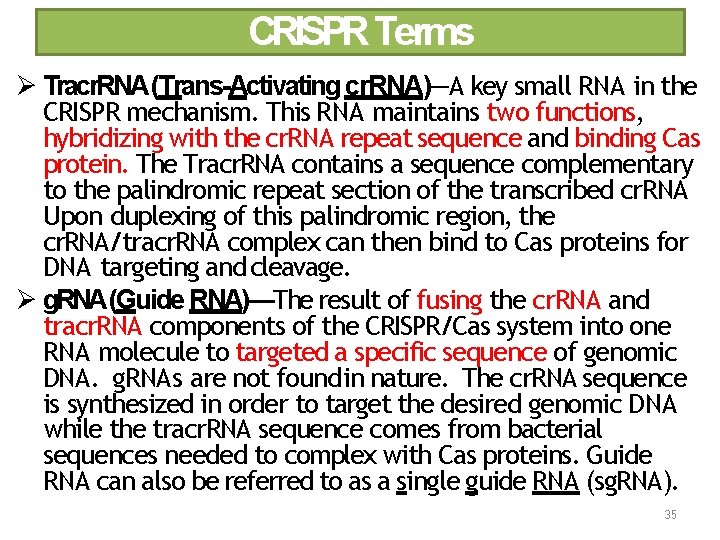
CRISPR Terms Tracr. RNA (Trans-Activating cr. RNA)—A key small RNA in the CRISPR mechanism. This RNA maintains two functions, hybridizing with the cr. RNA repeat sequence and binding Cas protein. The Tracr. RNA contains a sequence complementary to the palindromic repeat section of the transcribed cr. RNA Upon duplexing of this palindromic region, the cr. RNA/tracr. RNA complex can then bind to Cas proteins for DNA targeting and cleavage. g. RNA (Guide RNA)—The result of fusing the cr. RNA and tracr. RNA components of the CRISPR/Cas system into one RNA molecule to targeted a specific sequence of genomic DNA. g. RNAs are not found in nature. The cr. RNA sequence is synthesized in order to target the desired genomic DNA while the tracr. RNA sequence comes from bacterial sequences needed to complex with Cas proteins. Guide RNA can also be referred to as a single guide RNA (sg. RNA). 35
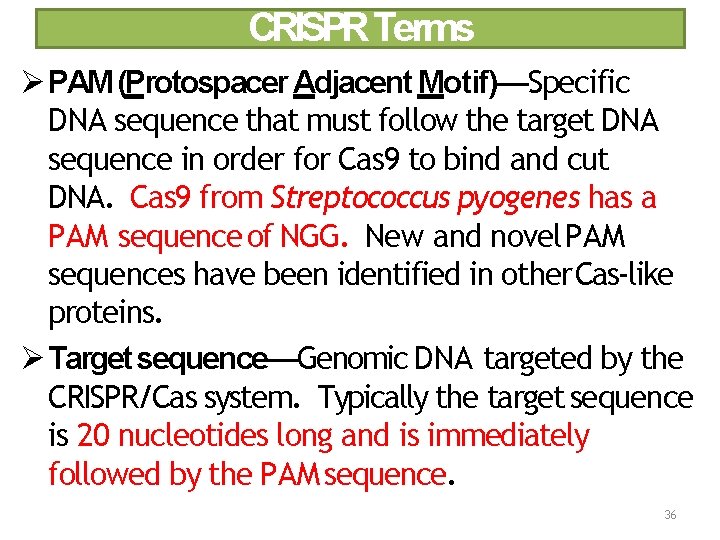
CRISPR Terms PAM (Protospacer Adjacent Motif)—Specific DNA sequence that must follow the target DNA sequence in order for Cas 9 to bind and cut DNA. Cas 9 from Streptococcus pyogenes has a PAM sequence of NGG. New and novel PAM sequences have been identified in other Cas‐like proteins. Target sequence—Genomic DNA targeted by the CRISPR/Cas system. Typically the target sequence is 20 nucleotides long and is immediately followed by the PAM sequence. 36
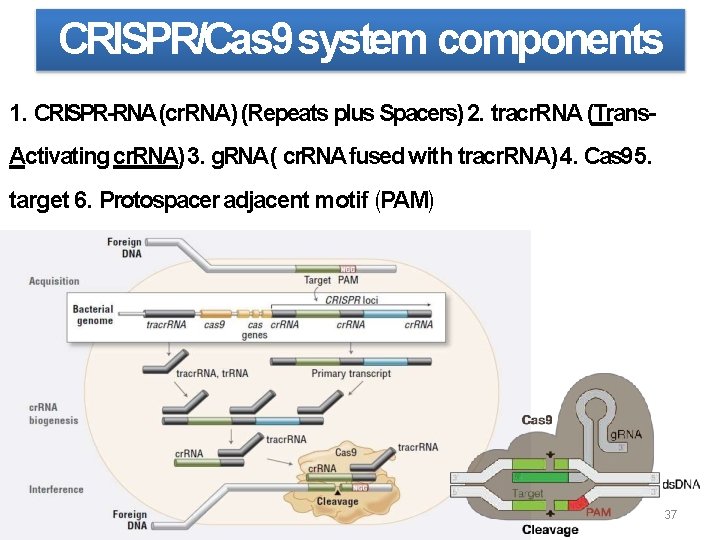
CRISPR/Cas 9 system components 1. CRISPR-RNA (cr. RNA) (Repeats plus Spacers) 2. tracr. RNA (Trans. Activating cr. RNA) 3. g. RNA( cr. RNA fused with tracr. RNA) 4. Cas 95. target 6. Protospacer adjacent motif (PAM) 3. trans-activating cr. RNA(tracr. RNA) 37
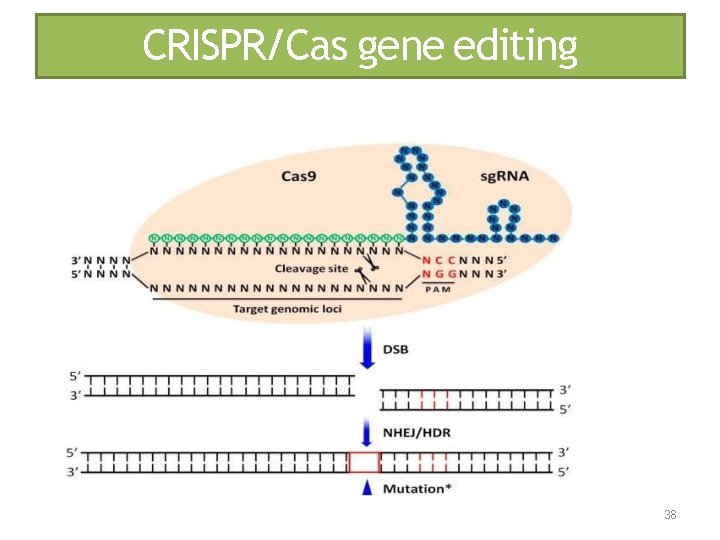
CRISPR/Cas gene editing 38
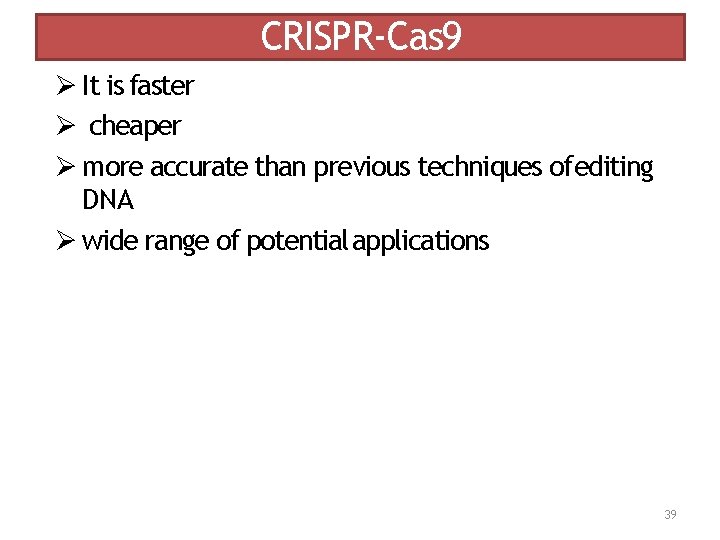
CRISPR‐Cas 9 It is faster cheaper more accurate than previous techniques of editing DNA wide range of potential applications 39
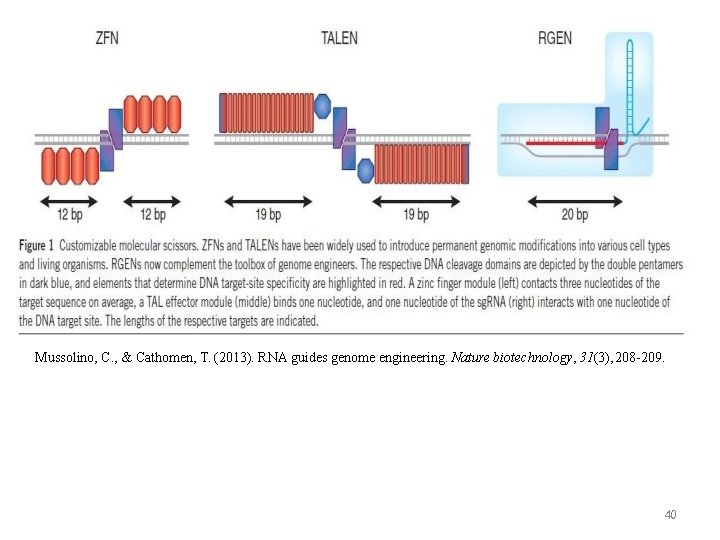
Mussolino, C. , & Cathomen, T. (2013). RNA guides genome engineering. Nature biotechnology, 31(3), 208 -209. 40
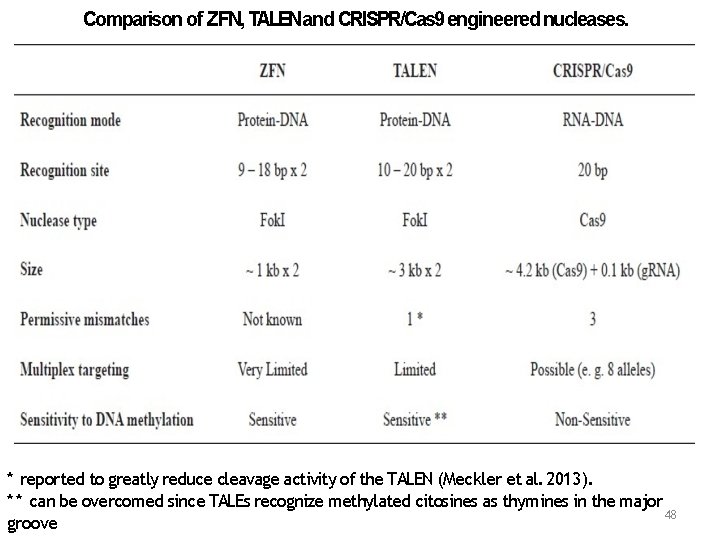
Comparison of ZFN, TALENand CRISPR/Cas 9 engineered nucleases. * reported to greatly reduce cleavage activity of the TALEN (Meckler et al. 2013). * * can be overcomed since TALEs recognize methylated citosines as thymines in the major 48 groove
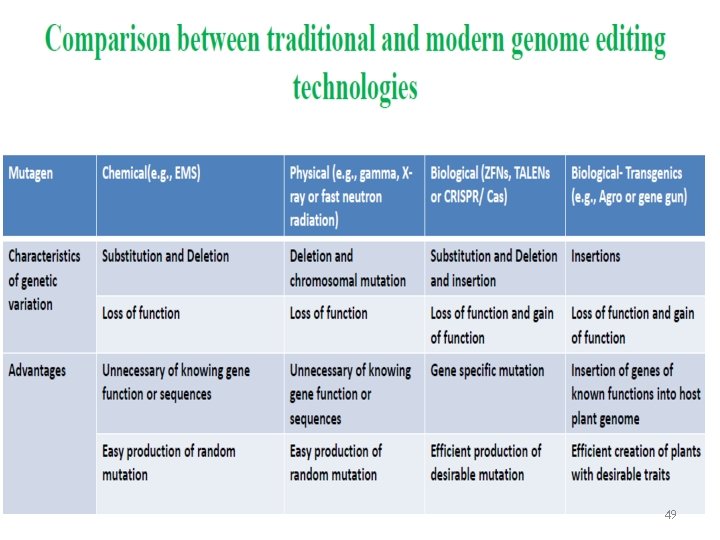
49
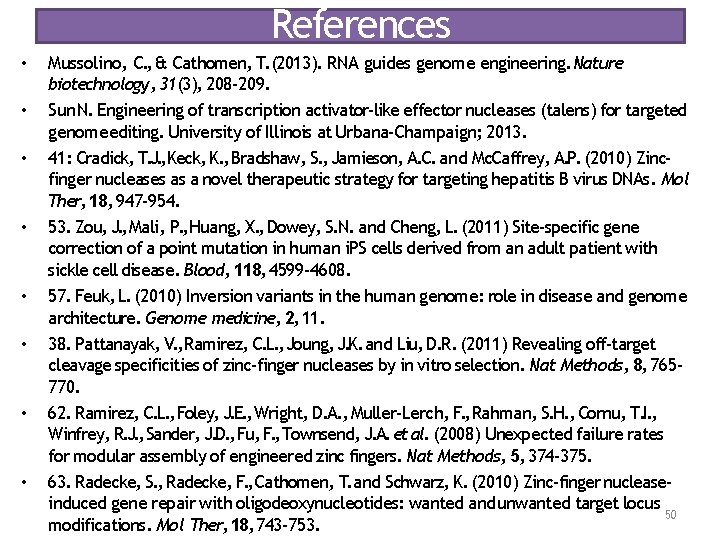
References • • Mussolino, C. , & Cathomen, T. (2013). RNA guides genome engineering. Nature biotechnology, 31(3), 208‐ 209. Sun N. Engineering of transcription activator‐like effector nucleases (talens) for targeted genome editing. University of Illinois at Urbana‐Champaign; 2013. 41: Cradick, T. J. , Keck, K. , Bradshaw, S. , Jamieson, A. C. and Mc. Caffrey, A. P. (2010) Zinc‐ finger nucleases as a novel therapeutic strategy for targeting hepatitis B virus DNAs. Mol Ther, 18, 947‐ 954. 53. Zou, J. , Mali, P. , Huang, X. , Dowey, S. N. and Cheng, L. (2011) Site‐specific gene correction of a point mutation in human i. PS cells derived from an adult patient with sickle cell disease. Blood, 118, 4599‐ 4608. 57. Feuk, L. (2010) Inversion variants in the human genome: role in disease and genome architecture. Genome medicine, 2, 11. 38. Pattanayak, V. , Ramirez, C. L. , Joung, J. K. and Liu, D. R. (2011) Revealing off‐target cleavage specificities of zinc‐finger nucleases by in vitro selection. Nat Methods, 8, 765‐ 770. 62. Ramirez, C. L. , Foley, J. E. , Wright, D. A. , Muller‐Lerch, F. , Rahman, S. H. , Cornu, T. I. , Winfrey, R. J. , Sander, J. D. , Fu, F. , Townsend, J. A. et al. (2008) Unexpected failure rates for modular assembly of engineered zinc fingers. Nat Methods, 5, 374‐ 375. 63. Radecke, S. , Radecke, F. , Cathomen, T. and Schwarz, K. (2010) Zinc‐finger nuclease‐ induced gene repair with oligodeoxynucleotides: wanted and unwanted target locus 50 modifications. Mol Ther, 18, 743‐ 753.
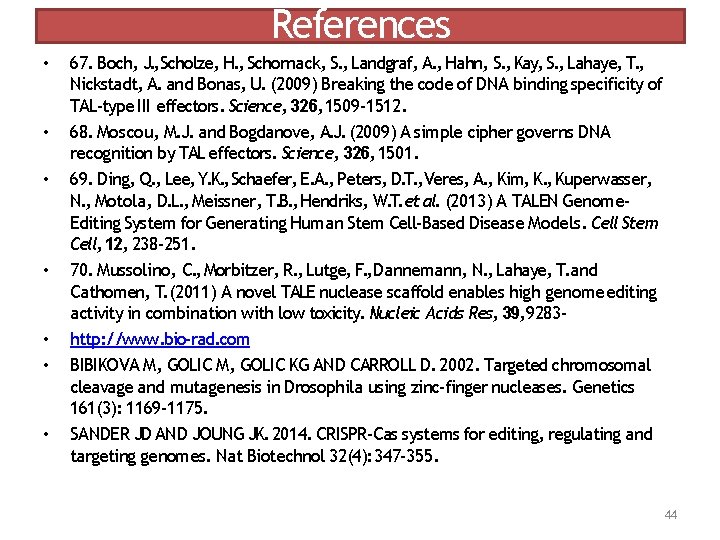
References • • 67. Boch, J. , Scholze, H. , Schornack, S. , Landgraf, A. , Hahn, S. , Kay, S. , Lahaye, T. , Nickstadt, A. and Bonas, U. (2009) Breaking the code of DNA binding specificity of TAL‐type III effectors. Science, 326, 1509‐ 1512. 68. Moscou, M. J. and Bogdanove, A. J. (2009) A simple cipher governs DNA recognition by TAL effectors. Science, 326, 1501. 69. Ding, Q. , Lee, Y. K. , Schaefer, E. A. , Peters, D. T. , Veres, A. , Kim, K. , Kuperwasser, N. , Motola, D. L. , Meissner, T. B. , Hendriks, W. T. et al. (2013) A TALEN Genome‐ Editing System for Generating Human Stem Cell‐Based Disease Models. Cell Stem Cell, 12, 238‐ 251. 70. Mussolino, C. , Morbitzer, R. , Lutge, F. , Dannemann, N. , Lahaye, T. and Cathomen, T. (2011) A novel TALE nuclease scaffold enables high genome editing activity in combination with low toxicity. Nucleic Acids Res, 39, 9283‐ http: //www. bio‐rad. com BIBIKOVA M, GOLIC KG AND CARROLL D. 2002. Targeted chromosomal cleavage and mutagenesis in Drosophila using zinc‐finger nucleases. Genetics 161(3): 1169‐ 1175. SANDER JD AND JOUNG JK. 2014. CRISPR‐Cas systems for editing, regulating and targeting genomes. Nat Biotechnol 32(4): 347‐ 355. 44
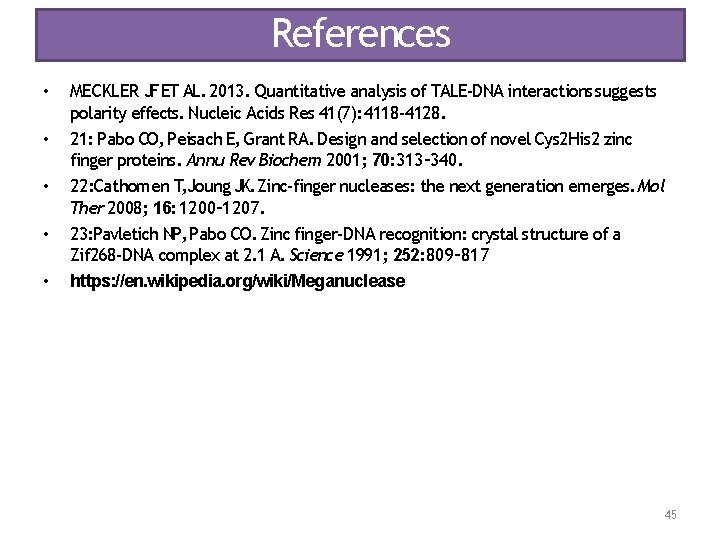
References • • • MECKLER JF ET AL. 2013. Quantitative analysis of TALE‐DNA interactions suggests polarity effects. Nucleic Acids Res 41(7): 4118‐ 4128. 21: Pabo CO, Peisach E, Grant RA. Design and selection of novel Cys 2 His 2 zinc finger proteins. Annu Rev Biochem 2001; 70: 313– 340. 22: Cathomen T, Joung JK. Zinc‐finger nucleases: the next generation emerges. Mol Ther 2008; 16: 1200– 1207. 23: Pavletich NP, Pabo CO. Zinc finger‐DNA recognition: crystal structure of a Zif 268‐DNA complex at 2. 1 A. Science 1991; 252: 809– 817 https: //en. wikipedia. org/wiki/Meganuclease 45
Semi-global alignment
Central editing in research methodology
Linear editing vs non linear editing
Patric bioinformatics
Savant genome browser
Biologists search the volumes of the human genome using
Gene finding
Strs and vntrs
Genome
Human genome project code
Plant genome research program
Dna
Genome adalah
Genome mapping
Artemis genome
Genome.gov
Mash genome
Genome assembly and annotation ppt
Chapter 14 the human genome
National human genome research institute
Epidna
Genome.gov
Perpartes
Genome identification
Genome klick
The human genome consists of
Human genome project source code
Igv genome
Genome project
Ap biol
Human genome size
National human genome research institute
Human genome project
Genome modification ustaz auni
Slidetodoc
Igv genome browser
Genome research limited
Human genome structure
Genome sequencing
History of sequencing
Stanford genome technology center
Sickle cell karyotype
Chapter 13 section 3 the human genome
Scale annotation pipeline
Alternate splicing
Turner syndrome