Structure of waveparticle interactions in nonlinear Alfvnic fluctuation
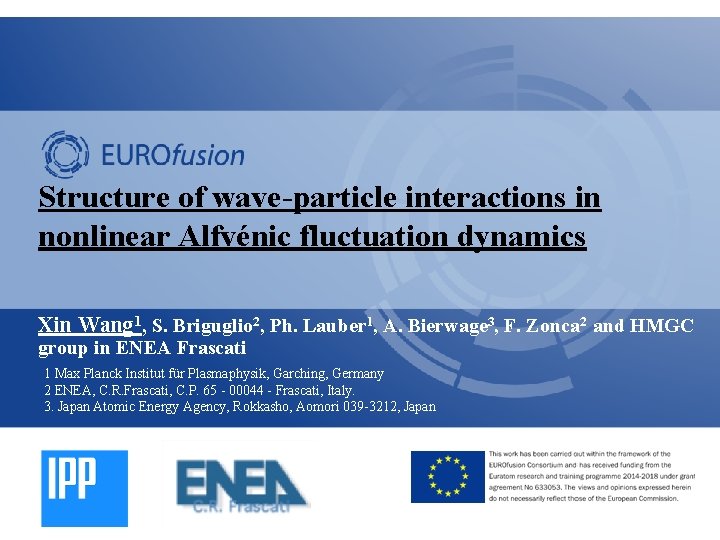
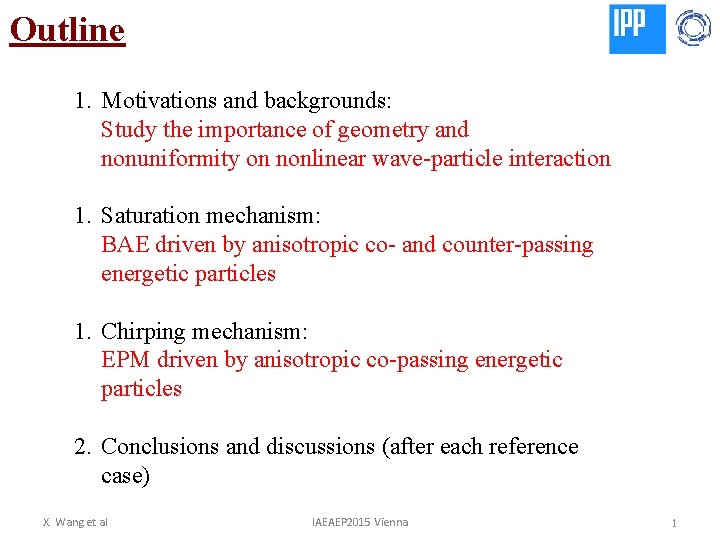
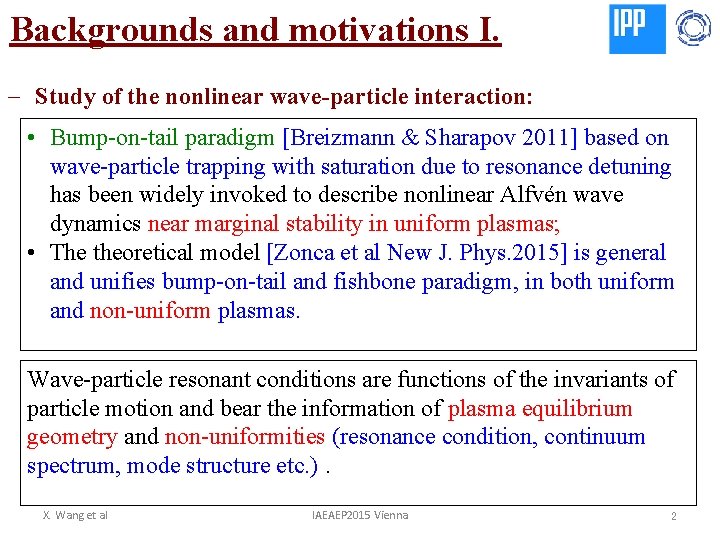
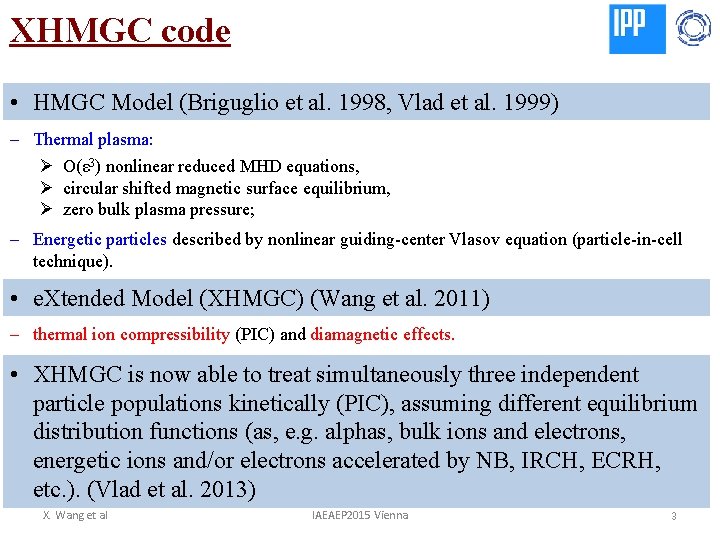
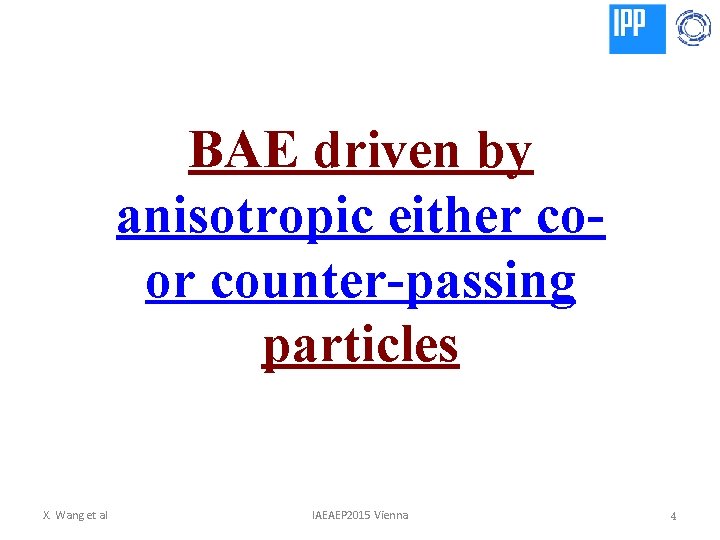
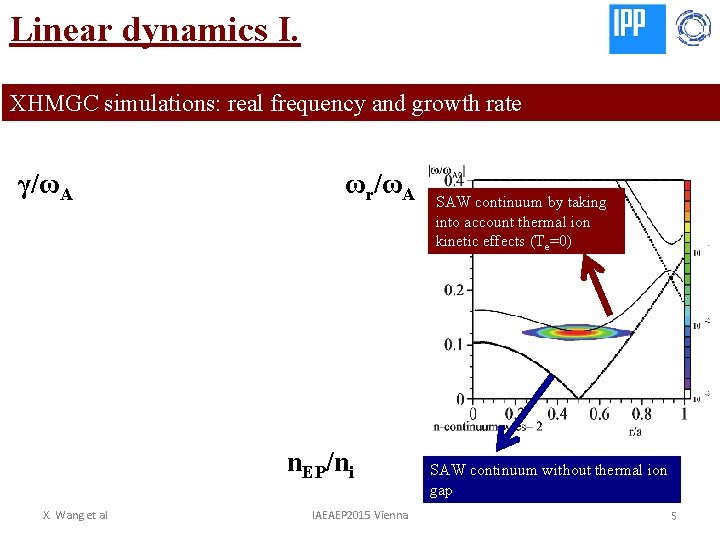
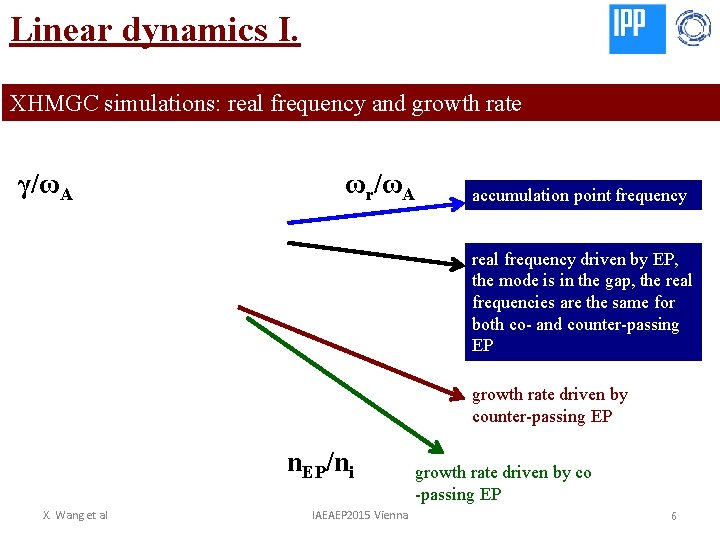
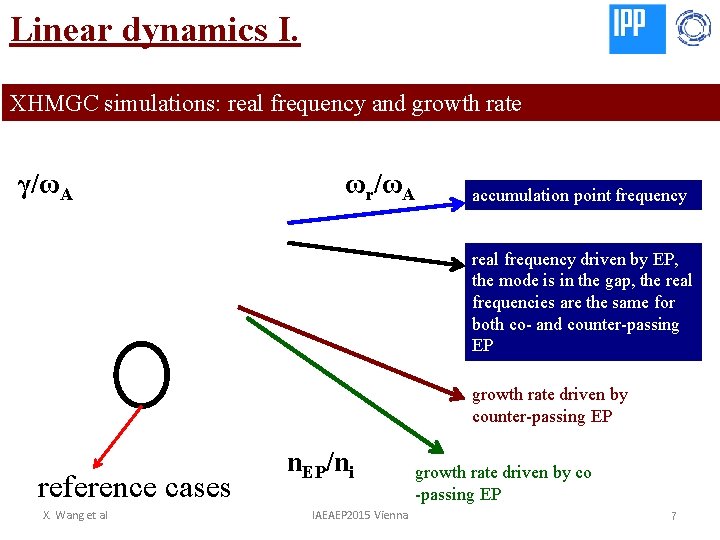
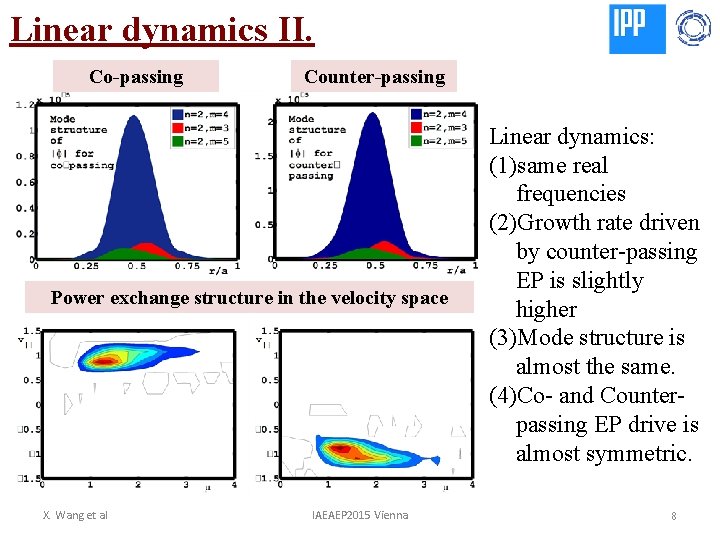
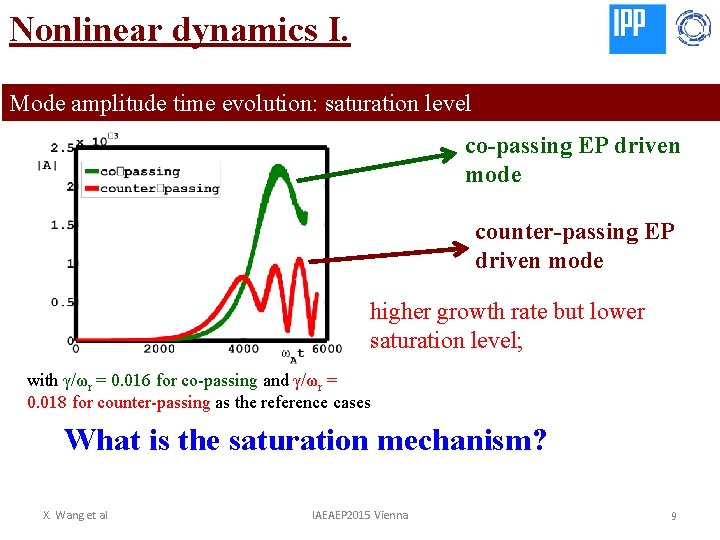
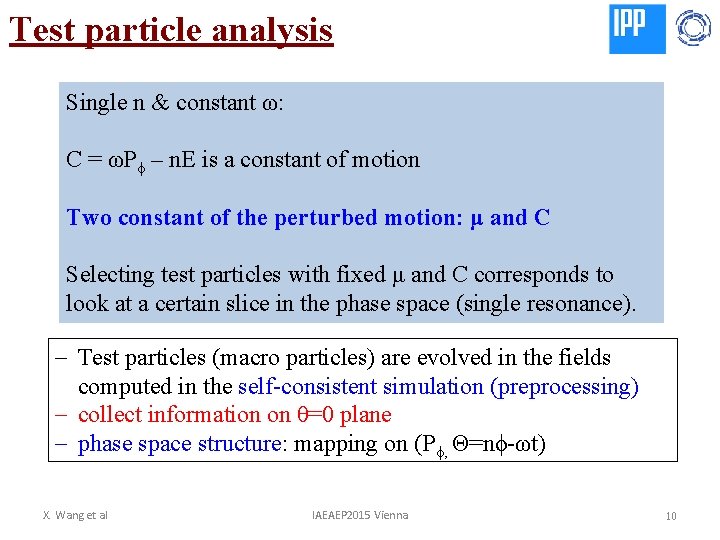
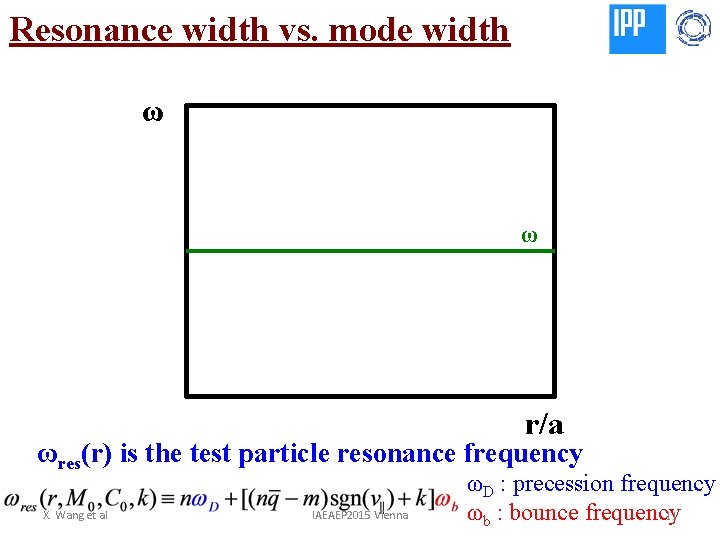
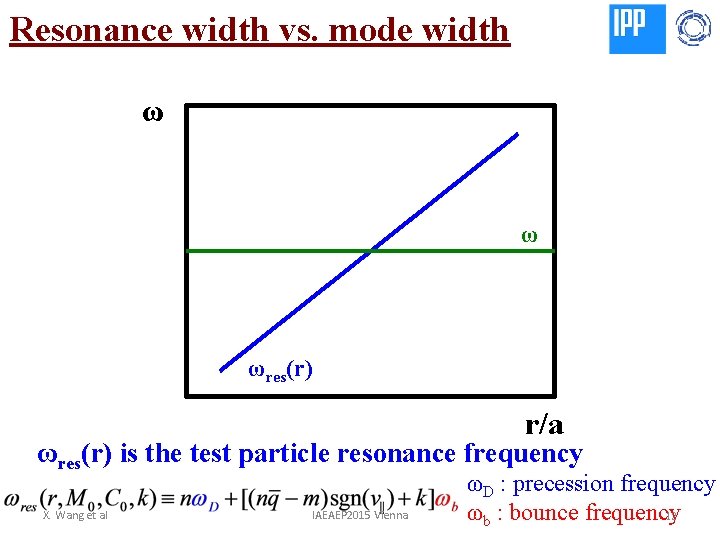
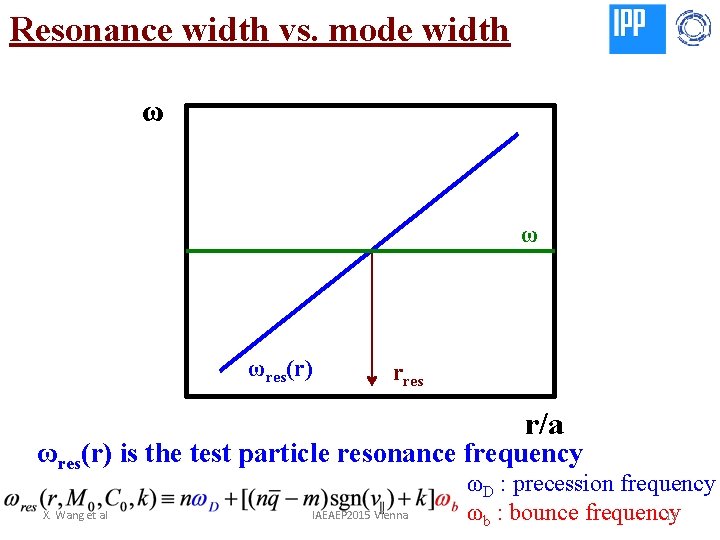
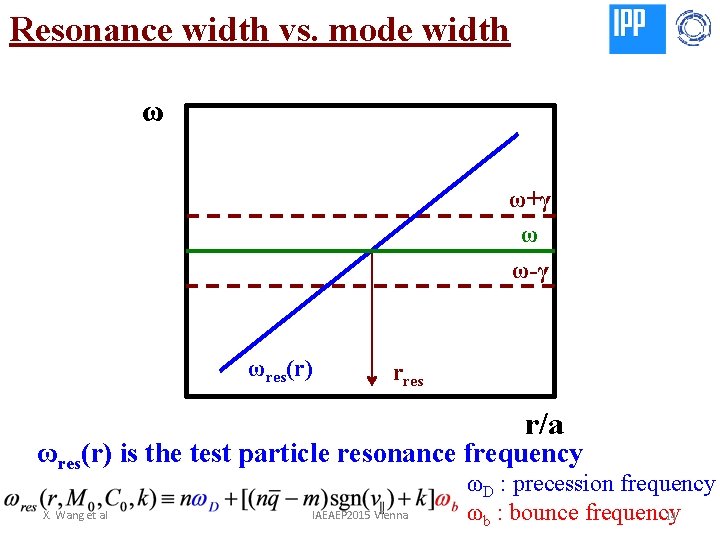
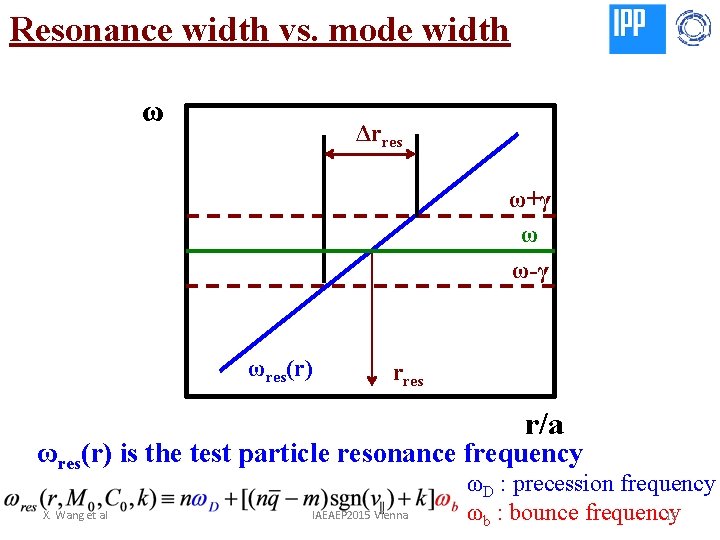
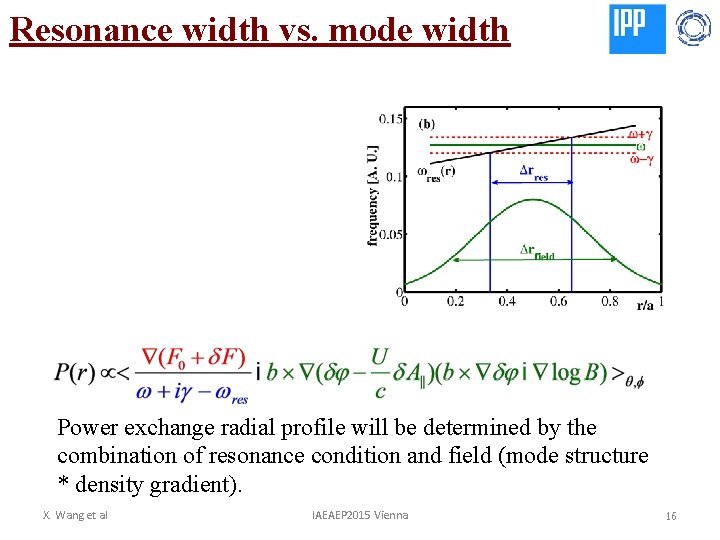
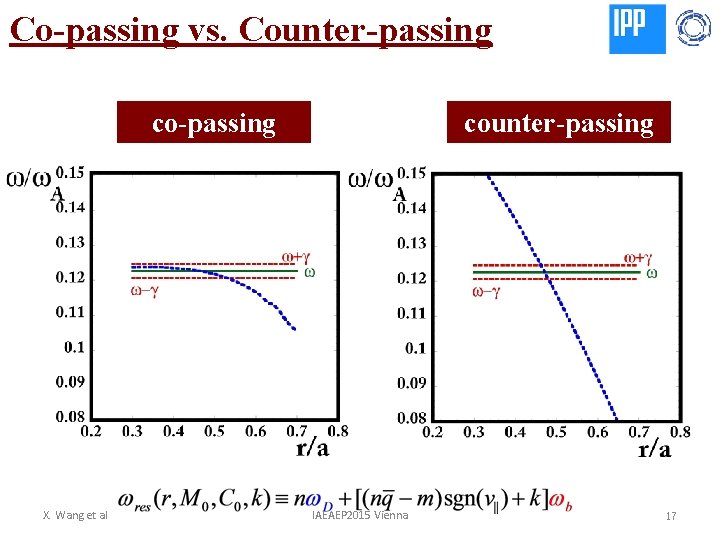
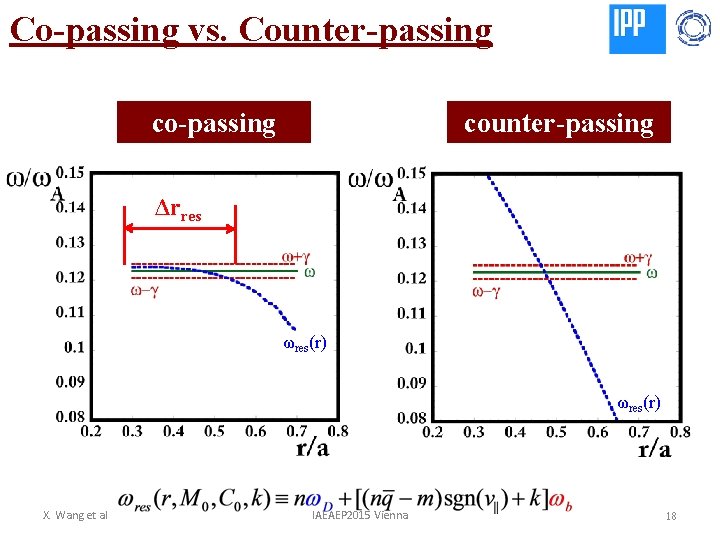
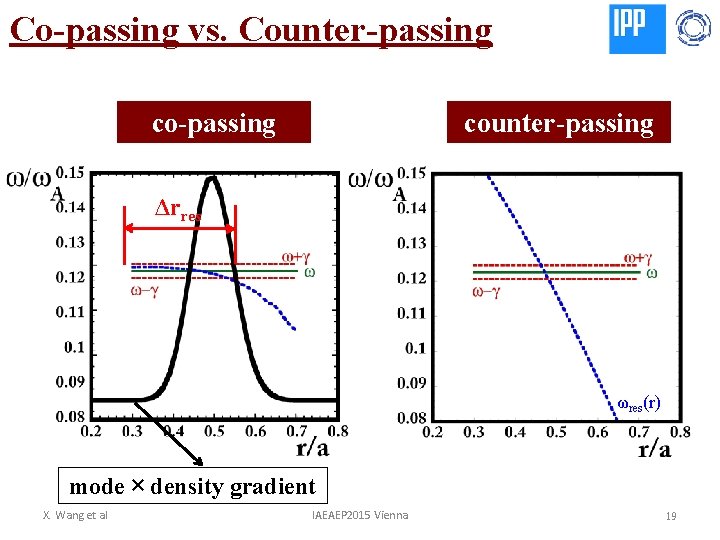
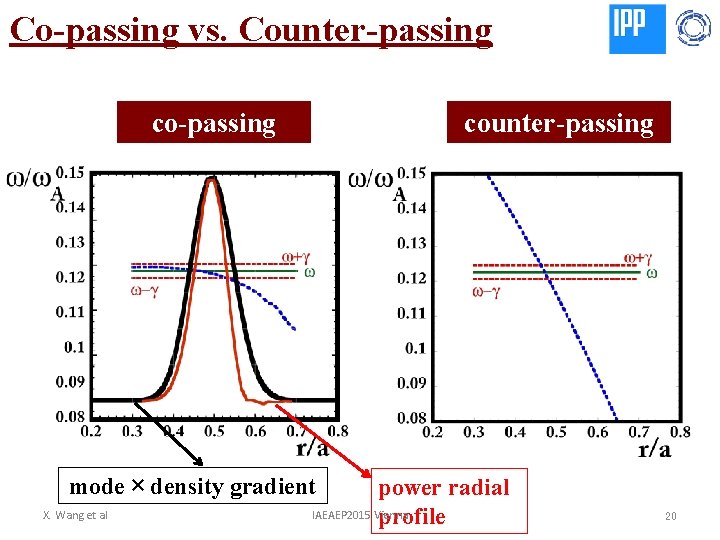
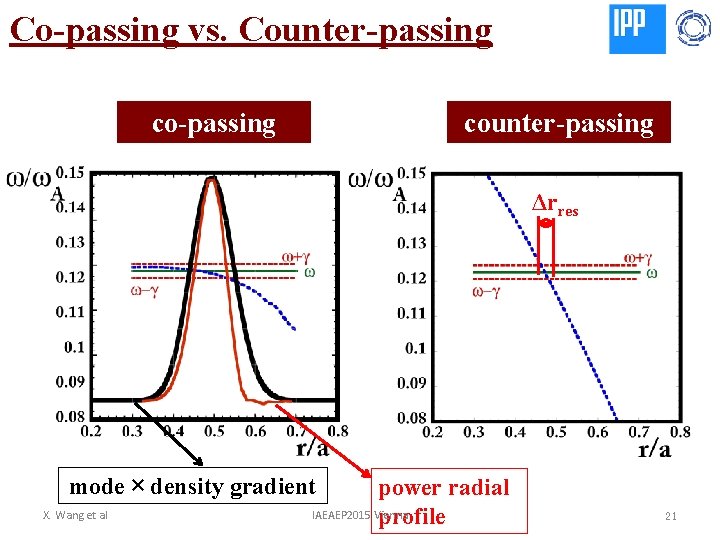
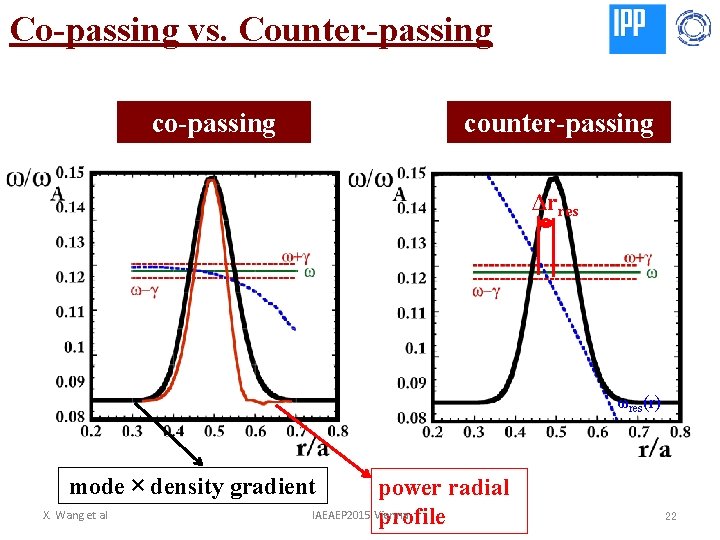
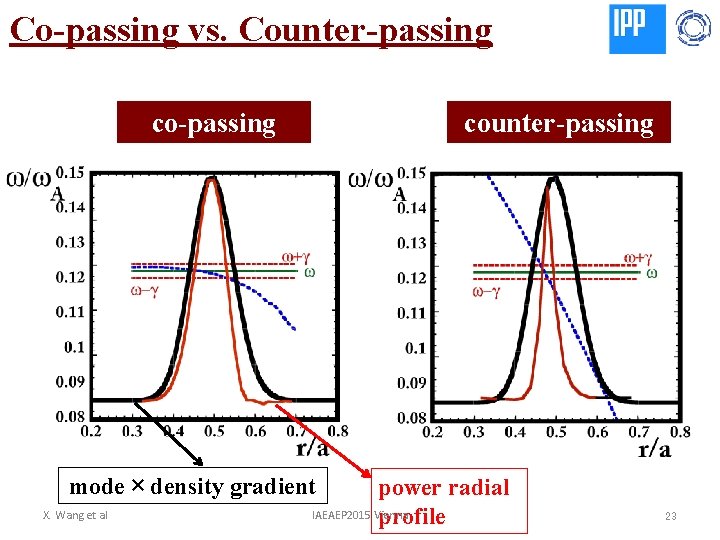
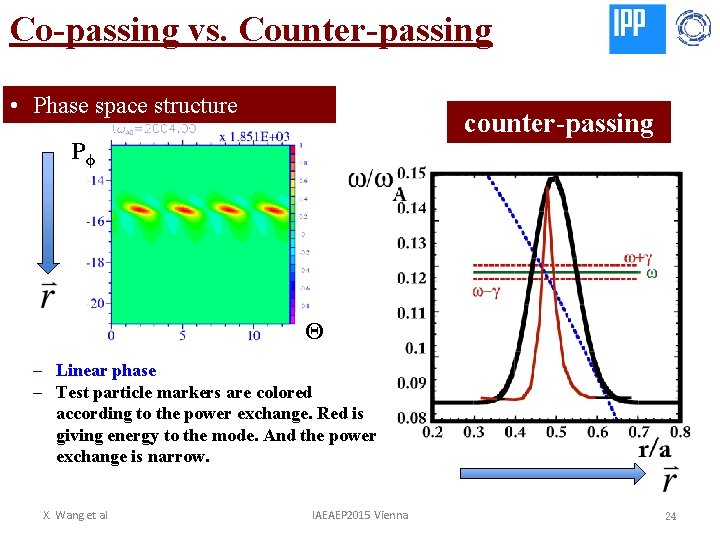
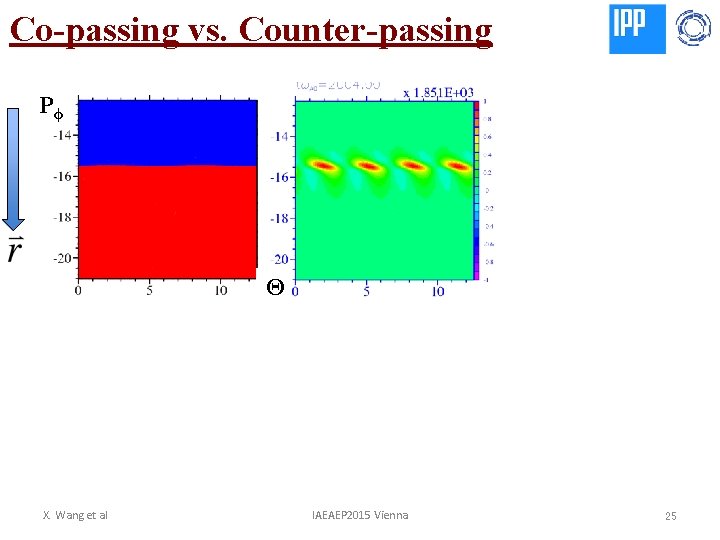
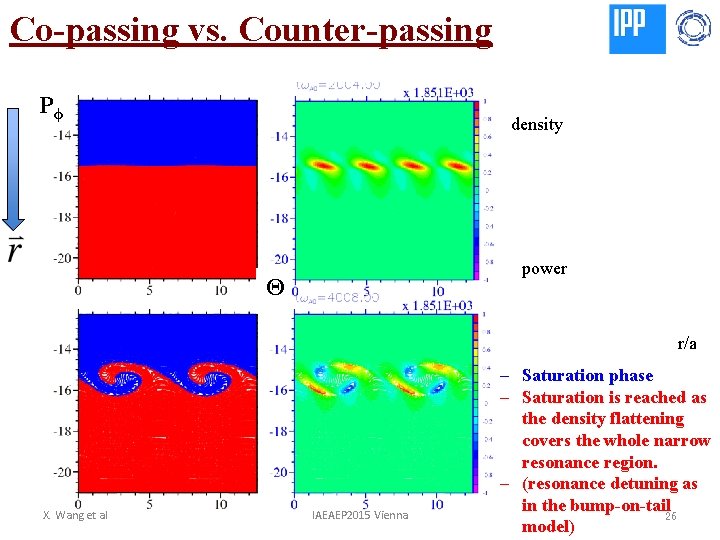
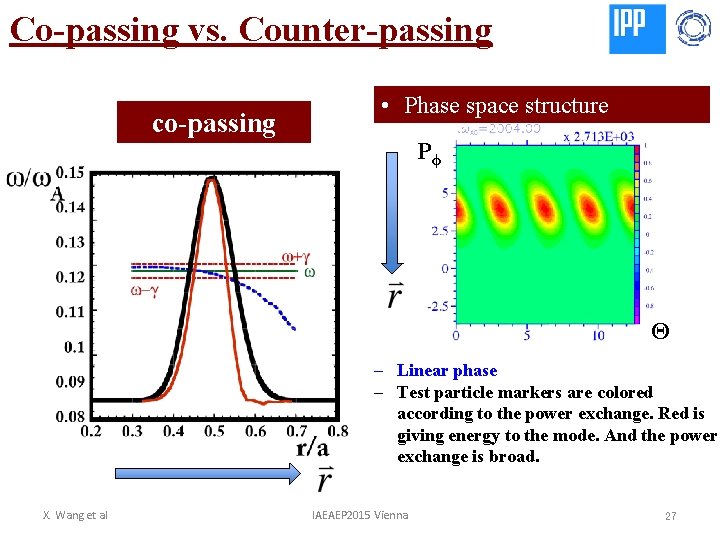
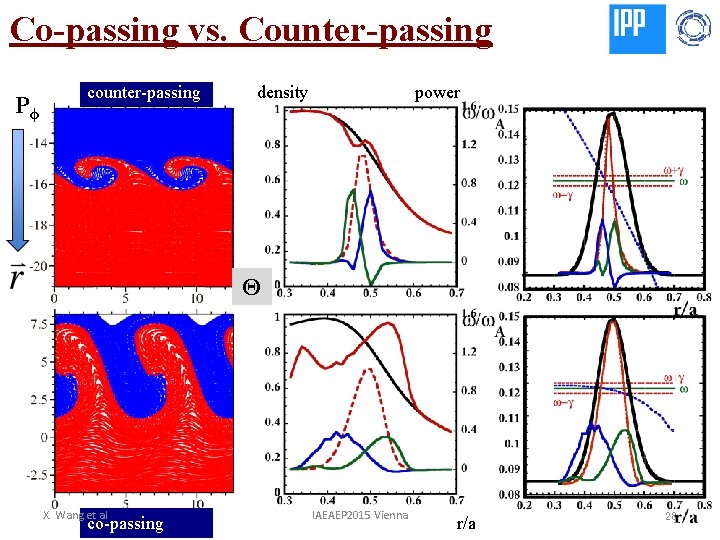
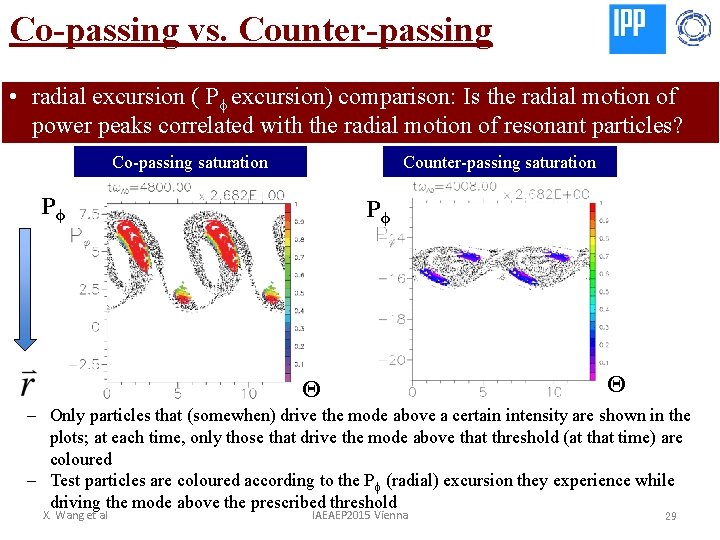
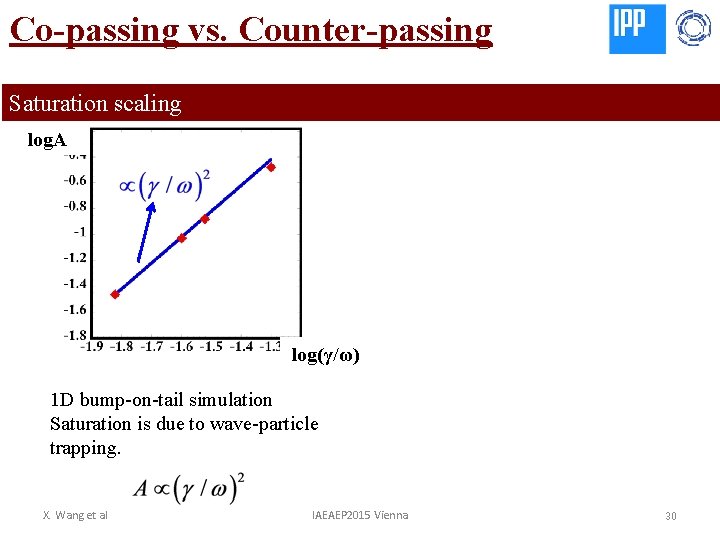
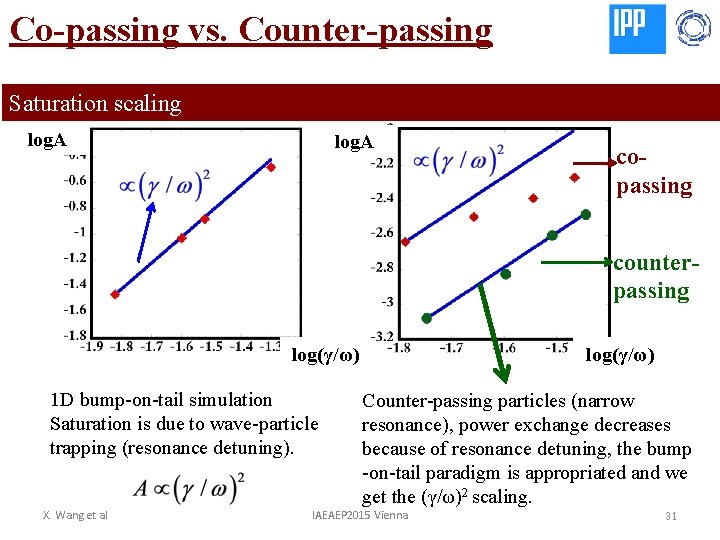
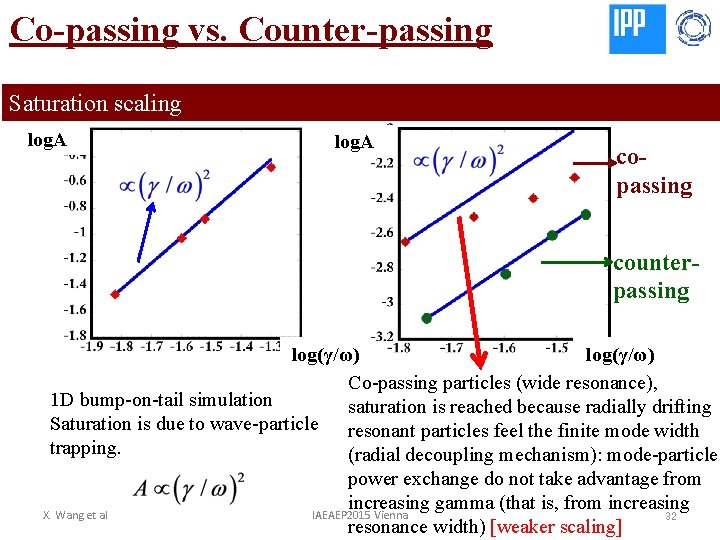
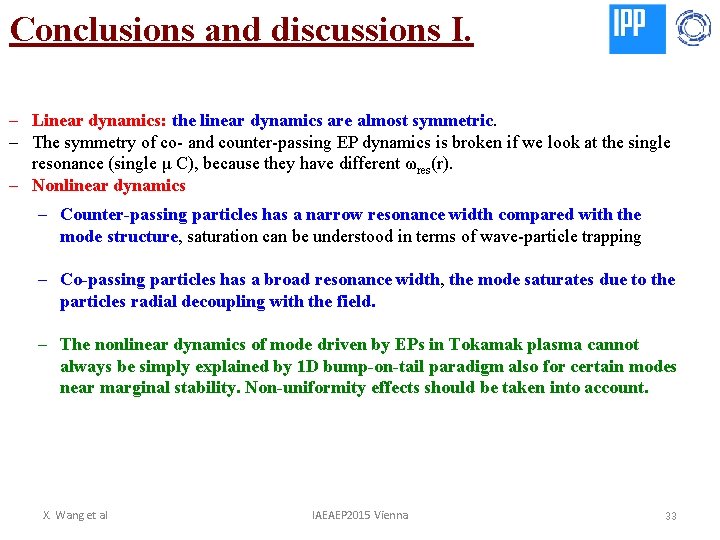
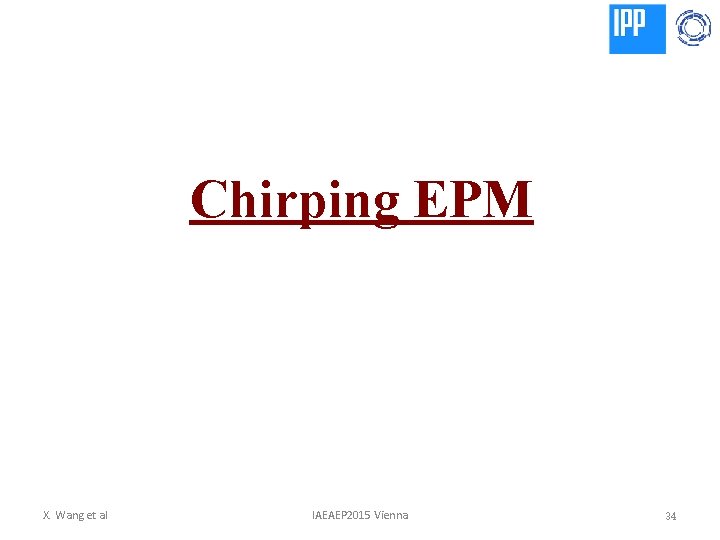
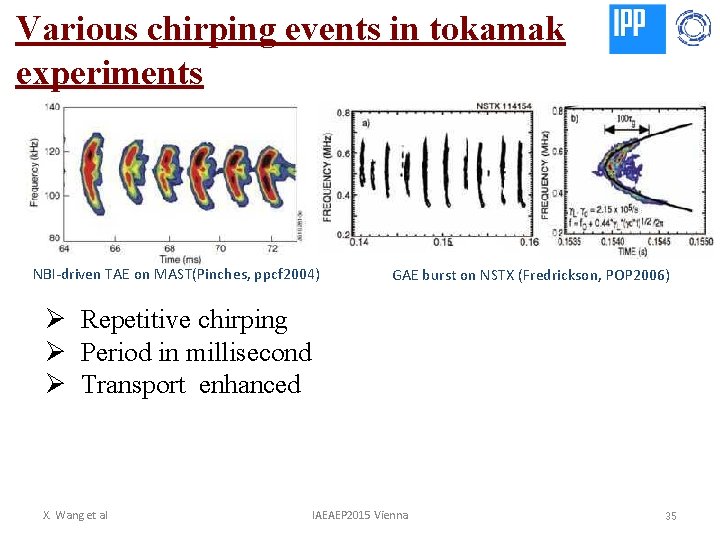
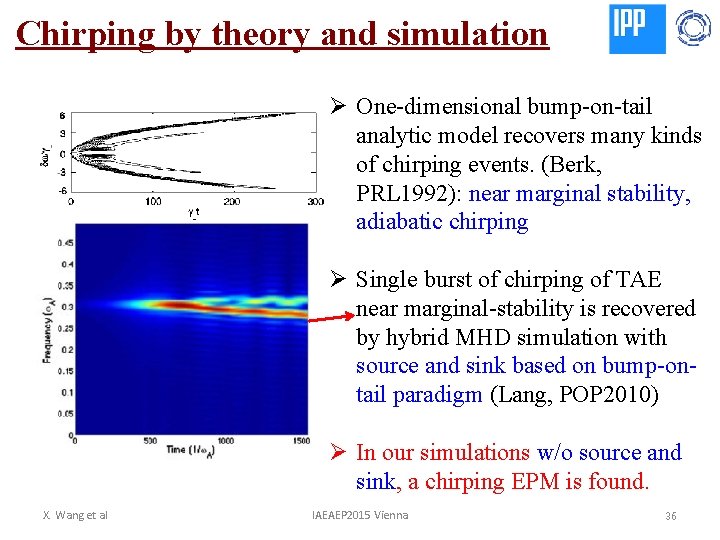
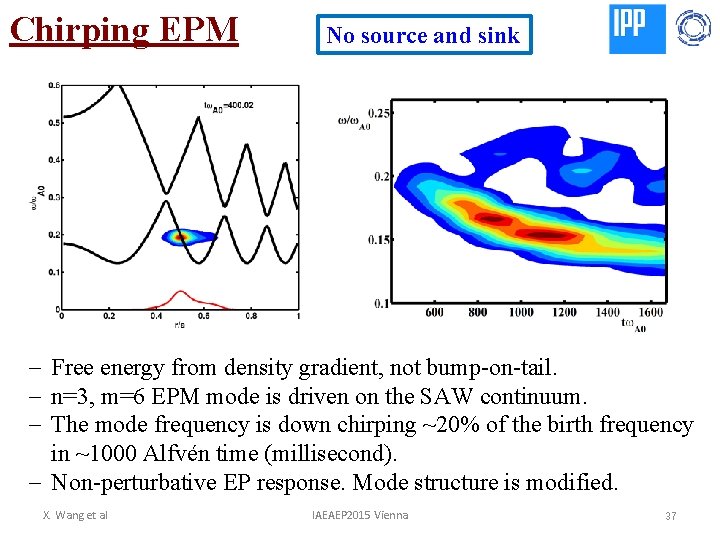
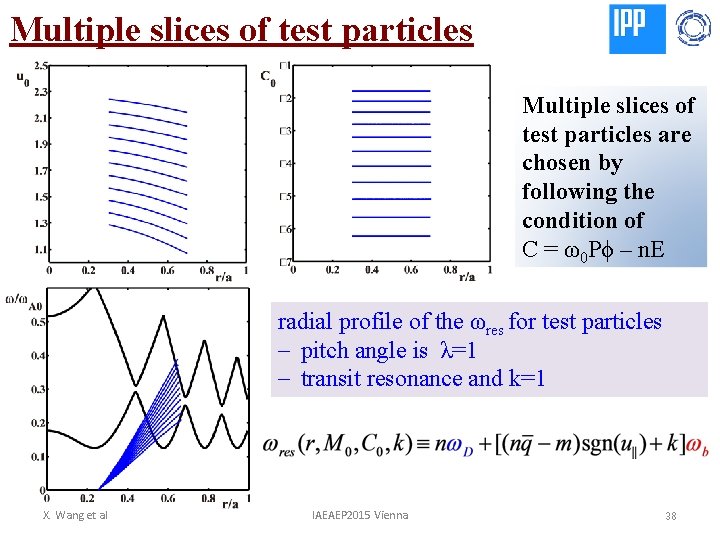
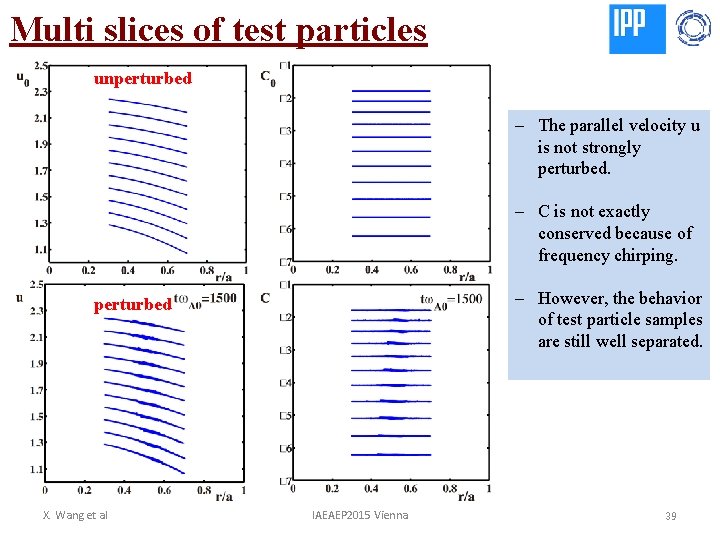
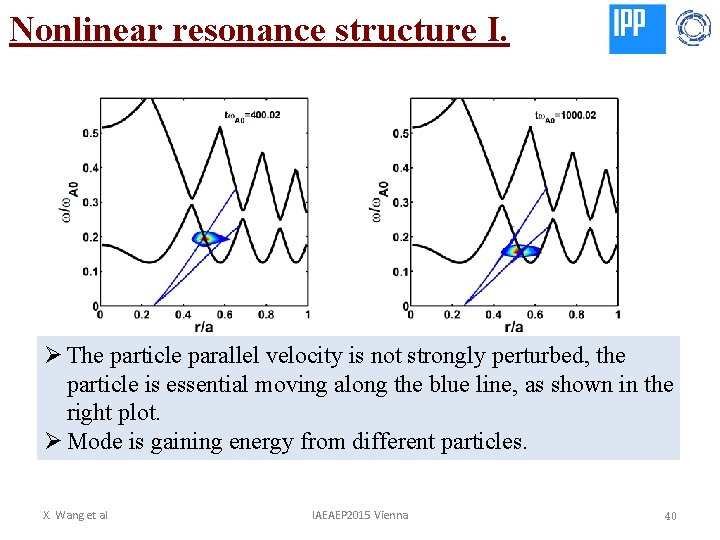
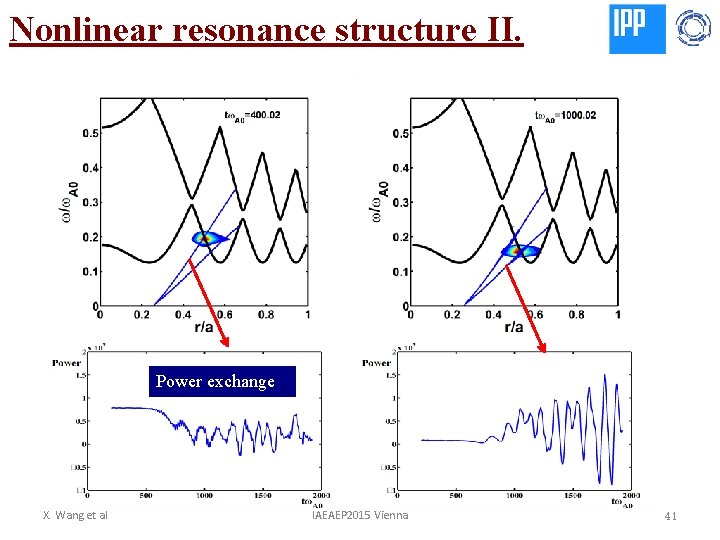
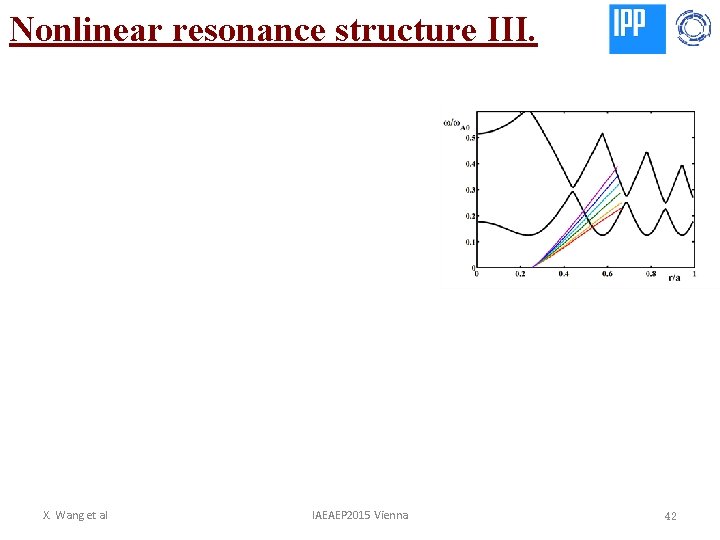
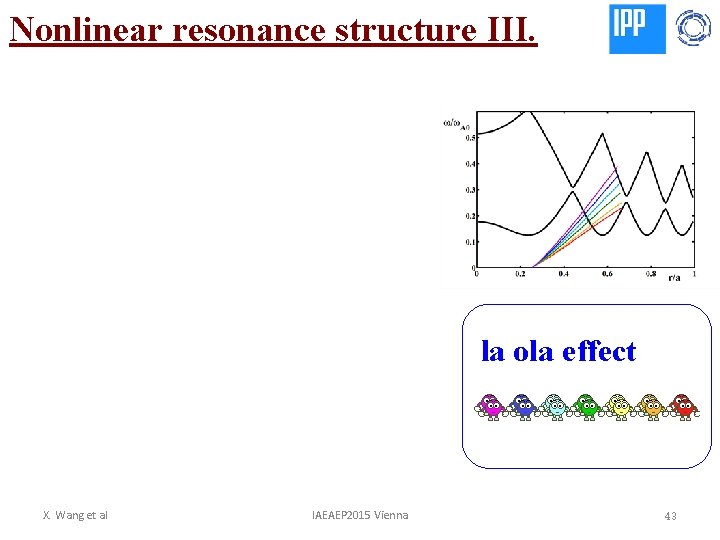
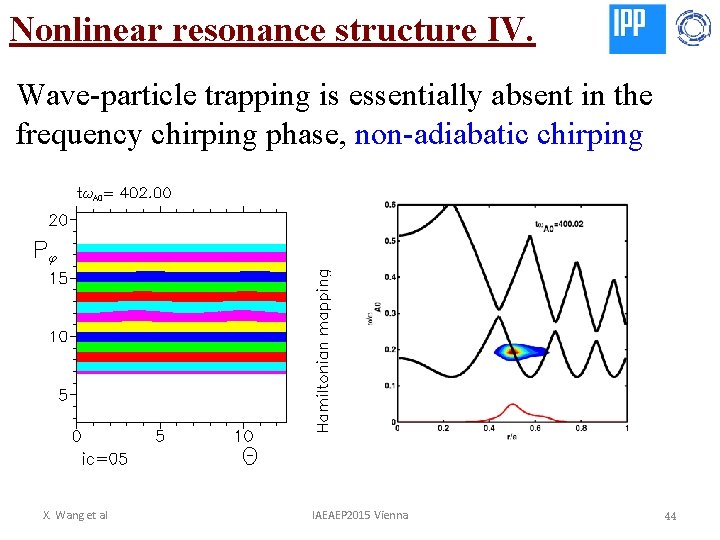
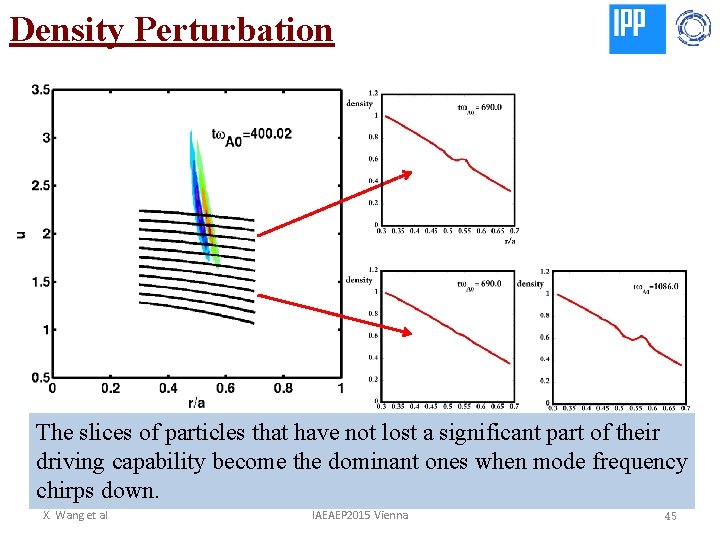
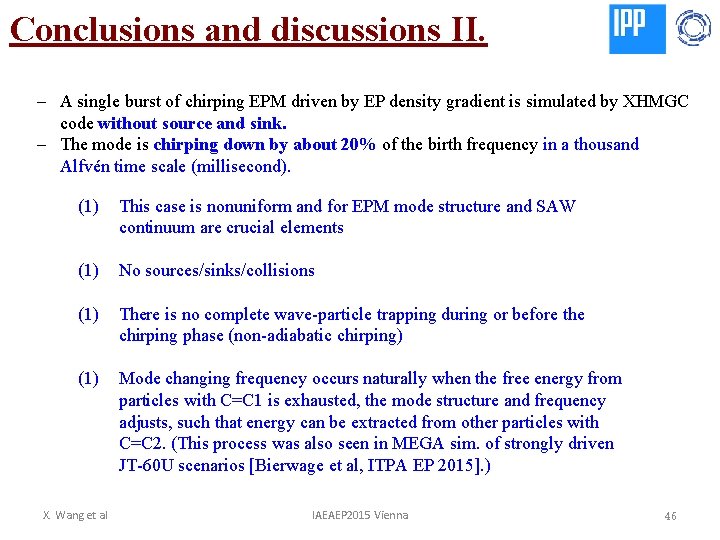
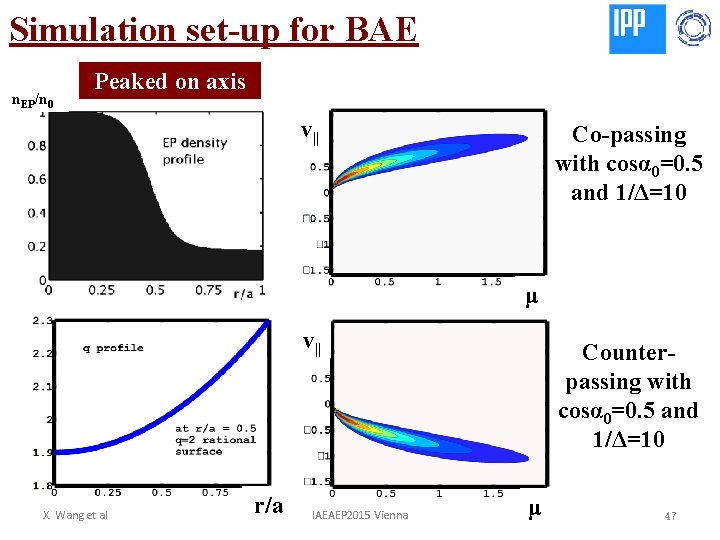
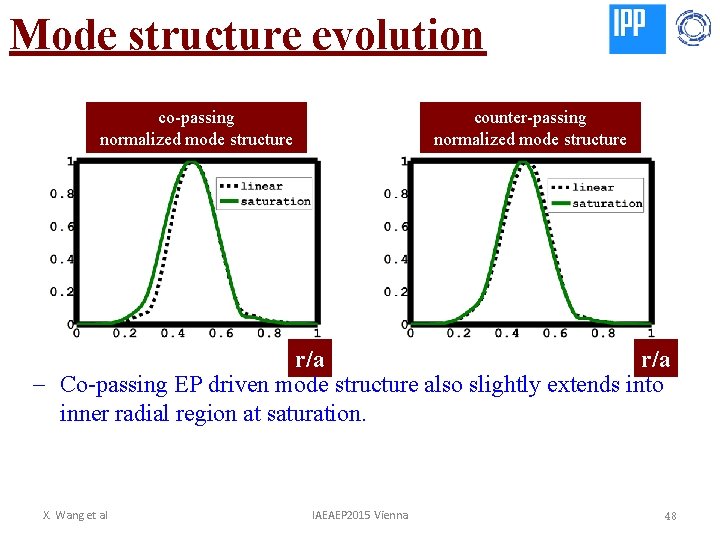
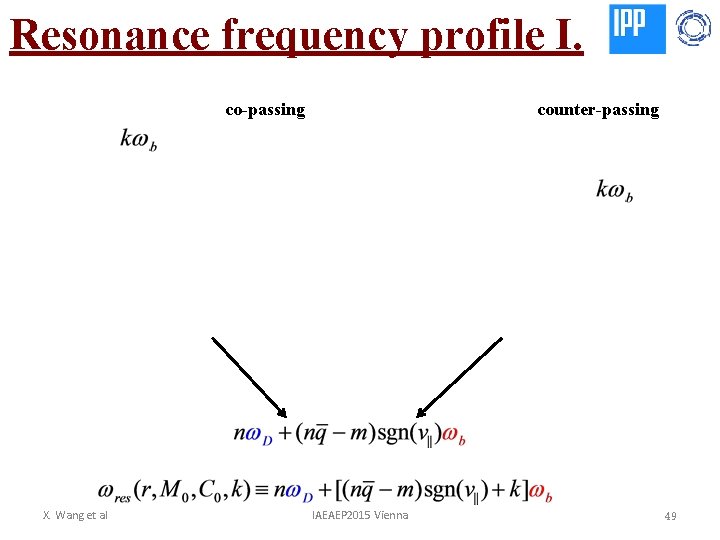
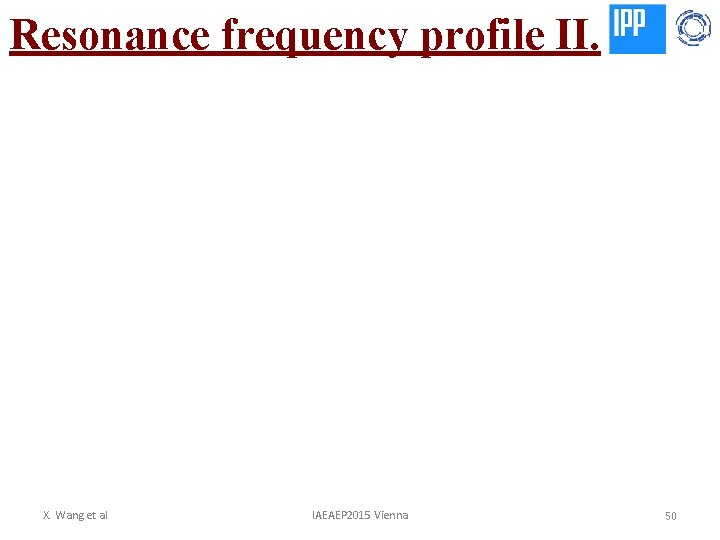
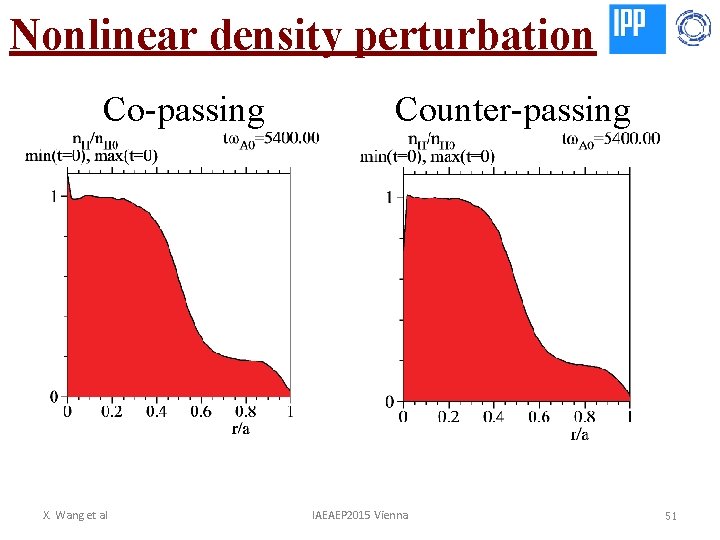
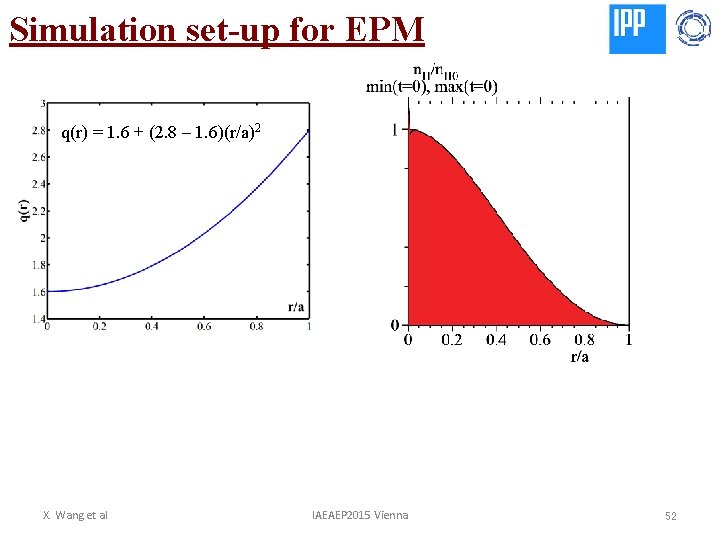
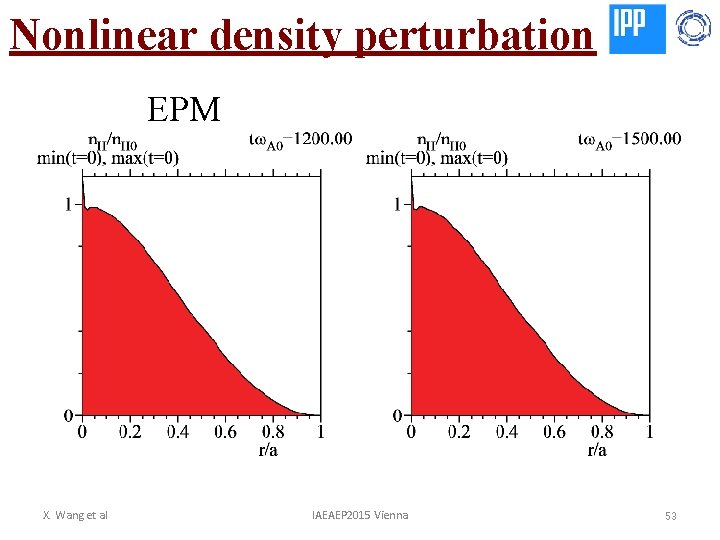
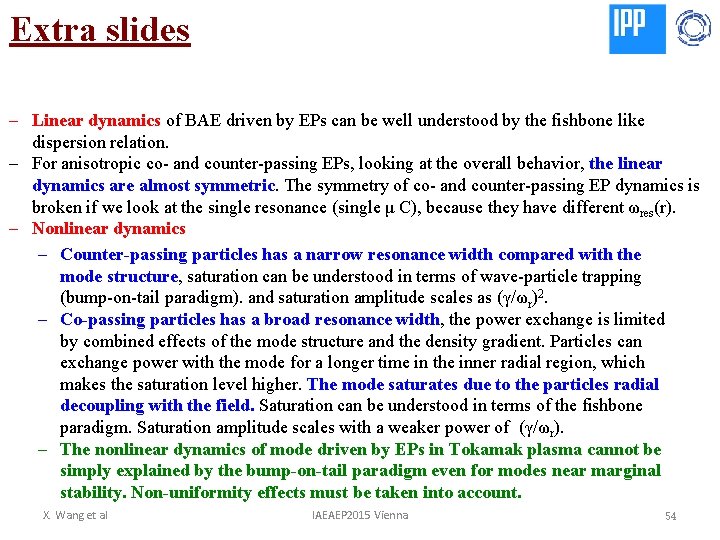
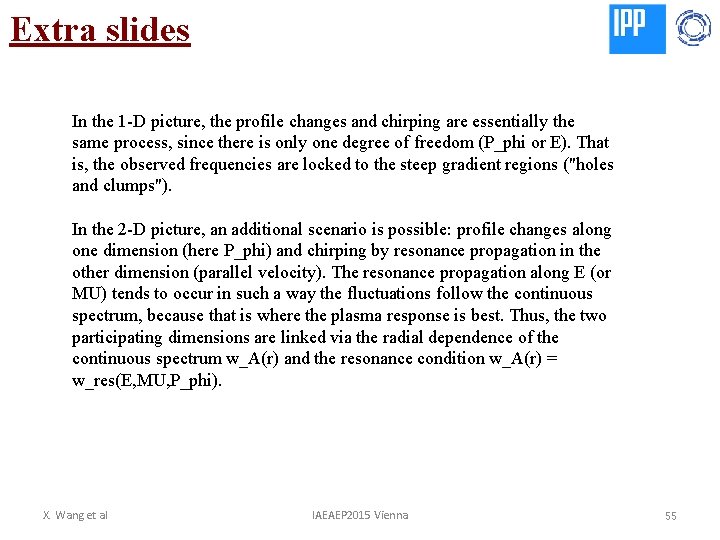
- Slides: 56
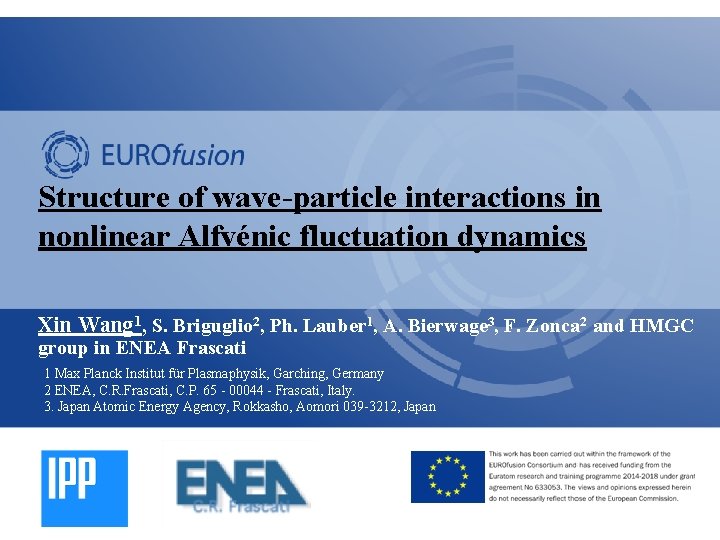
Structure of wave-particle interactions in nonlinear Alfvénic fluctuation dynamics Xin Wang 1, S. Briguglio 2, Ph. Lauber 1, A. Bierwage 3, F. Zonca 2 and HMGC group in ENEA Frascati 1 Max Planck Institut für Plasmaphysik, Garching, Germany 2 ENEA, C. R. Frascati, C. P. 65 - 00044 - Frascati, Italy. 3. Japan Atomic Energy Agency, Rokkasho, Aomori 039 -3212, Japan
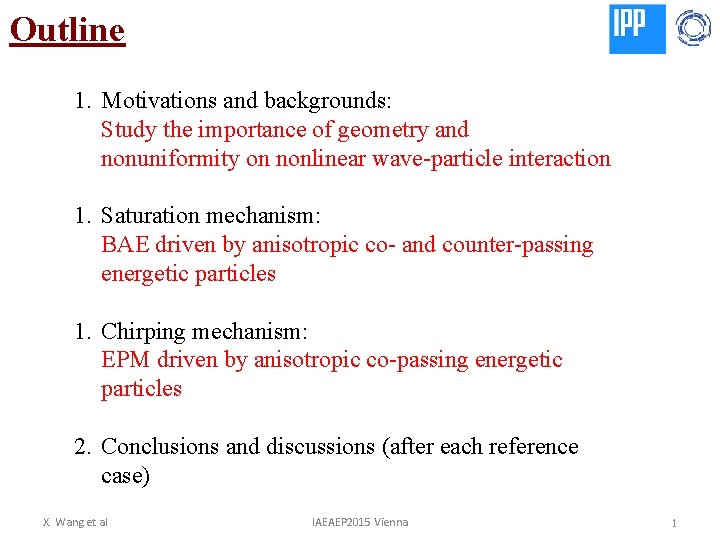
Outline 1. Motivations and backgrounds: Study the importance of geometry and nonuniformity on nonlinear wave-particle interaction 1. Saturation mechanism: BAE driven by anisotropic co- and counter-passing energetic particles 1. Chirping mechanism: EPM driven by anisotropic co-passing energetic particles 2. Conclusions and discussions (after each reference case) X. Wang et al IAEAEP 2015 Vienna 1
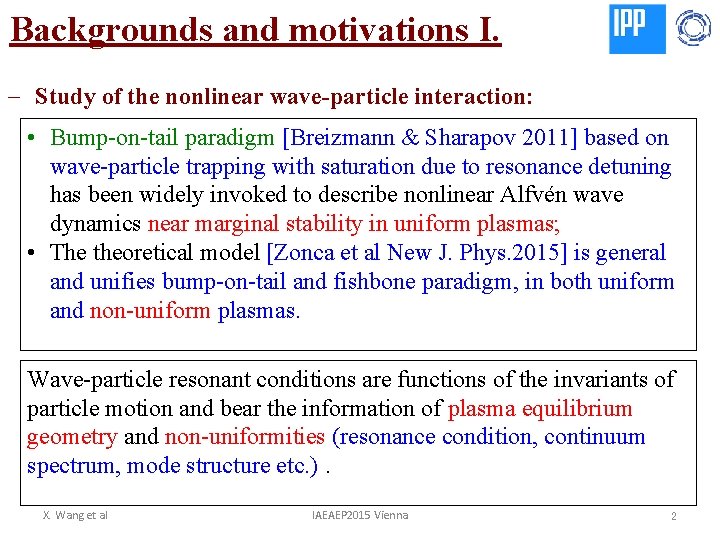
Backgrounds and motivations I. - Study of the nonlinear wave-particle interaction: • Bump-on-tail paradigm [Breizmann & Sharapov 2011] based on wave-particle trapping with saturation due to resonance detuning has been widely invoked to describe nonlinear Alfvén wave dynamics near marginal stability in uniform plasmas; • The theoretical model [Zonca et al New J. Phys. 2015] is general and unifies bump-on-tail and fishbone paradigm, in both uniform and non-uniform plasmas. Wave-particle resonant conditions are functions of the invariants of particle motion and bear the information of plasma equilibrium geometry and non-uniformities (resonance condition, continuum spectrum, mode structure etc. ). X. Wang et al IAEAEP 2015 Vienna 2
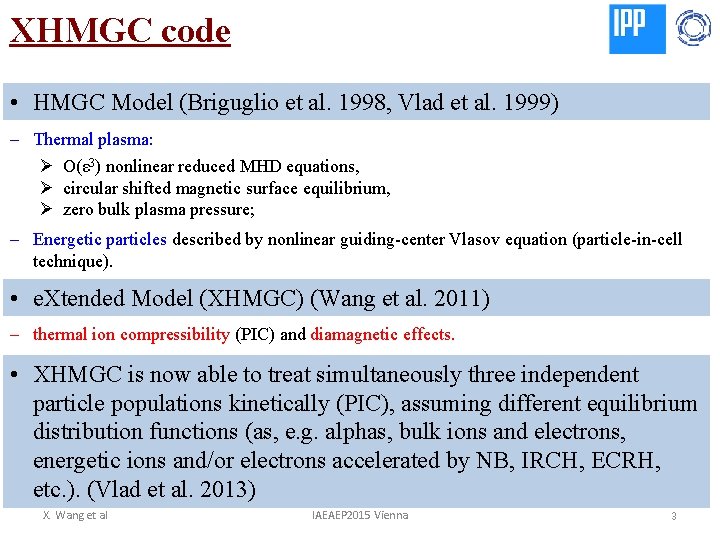
XHMGC code • HMGC Model (Briguglio et al. 1998, Vlad et al. 1999) - Thermal plasma: Ø O(ε 3) nonlinear reduced MHD equations, Ø circular shifted magnetic surface equilibrium, Ø zero bulk plasma pressure; - Energetic particles described by nonlinear guiding-center Vlasov equation (particle-in-cell technique). • e. Xtended Model (XHMGC) (Wang et al. 2011) - thermal ion compressibility (PIC) and diamagnetic effects. • XHMGC is now able to treat simultaneously three independent particle populations kinetically (PIC), assuming different equilibrium distribution functions (as, e. g. alphas, bulk ions and electrons, energetic ions and/or electrons accelerated by NB, IRCH, ECRH, etc. ). (Vlad et al. 2013) X. Wang et al IAEAEP 2015 Vienna 3
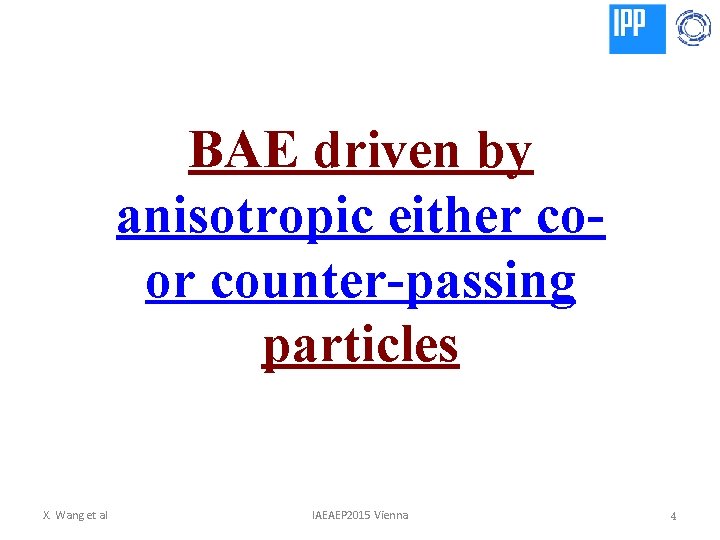
BAE driven by anisotropic either coor counter-passing particles X. Wang et al IAEAEP 2015 Vienna 4
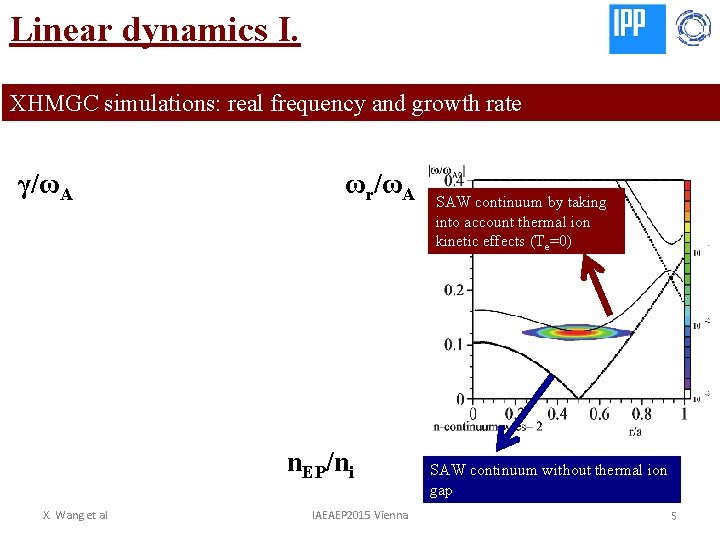
Linear dynamics I. XHMGC simulations: real frequency and growth rate γ/ωA ωr/ωA n. EP/ni X. Wang et al IAEAEP 2015 Vienna SAW continuum by taking into account thermal ion kinetic effects (Te=0) SAW continuum without thermal ion gap 5
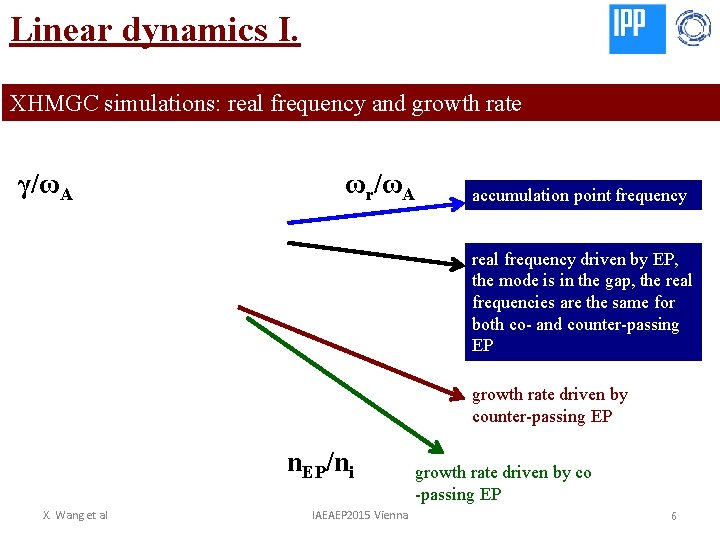
Linear dynamics I. XHMGC simulations: real frequency and growth rate γ/ωA ωr/ωA accumulation point frequency real frequency driven by EP, the mode is in the gap, the real frequencies are the same for both co- and counter-passing EP growth rate driven by counter-passing EP n. EP/ni X. Wang et al IAEAEP 2015 Vienna growth rate driven by co -passing EP 6
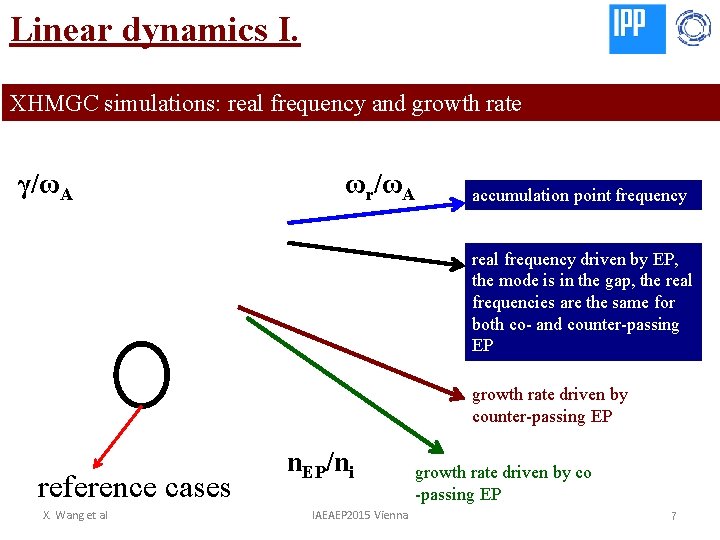
Linear dynamics I. XHMGC simulations: real frequency and growth rate γ/ωA ωr/ωA accumulation point frequency real frequency driven by EP, the mode is in the gap, the real frequencies are the same for both co- and counter-passing EP growth rate driven by counter-passing EP reference cases X. Wang et al n. EP/ni IAEAEP 2015 Vienna growth rate driven by co -passing EP 7
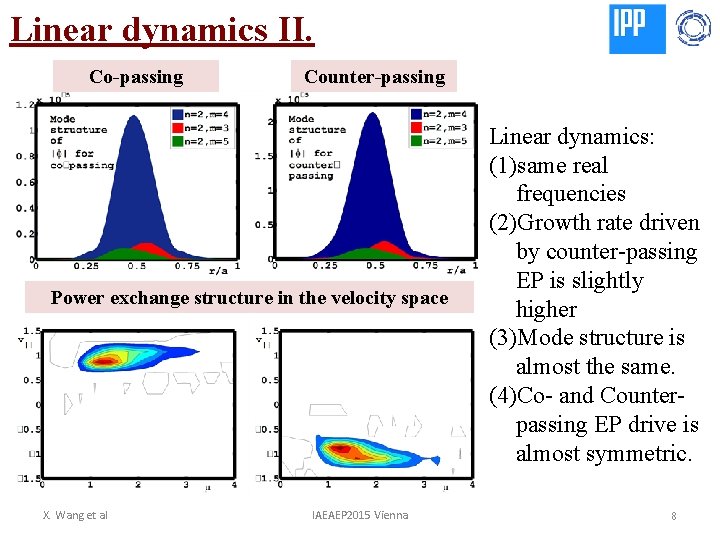
Linear dynamics II. Co-passing Counter-passing Power exchange structure in the velocity space X. Wang et al IAEAEP 2015 Vienna Linear dynamics: (1)same real frequencies (2)Growth rate driven by counter-passing EP is slightly higher (3)Mode structure is almost the same. (4)Co- and Counterpassing EP drive is almost symmetric. 8
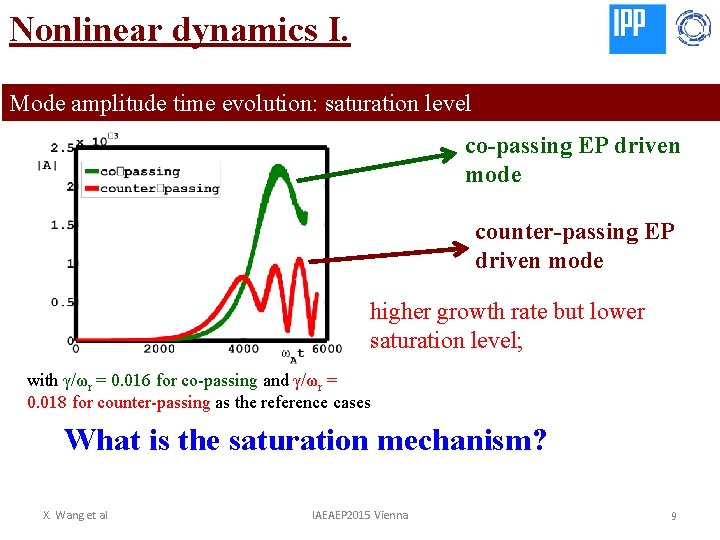
Nonlinear dynamics I. Mode amplitude time evolution: saturation level co-passing EP driven mode counter-passing EP driven mode higher growth rate but lower saturation level; with γ/ωr = 0. 016 for co-passing and γ/ωr = 0. 018 for counter-passing as the reference cases What is the saturation mechanism? X. Wang et al IAEAEP 2015 Vienna 9
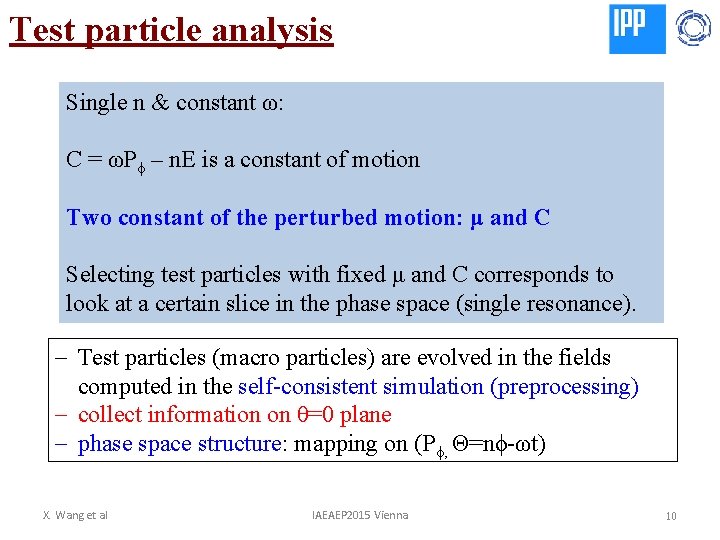
Test particle analysis Single n & constant ω: C = ωPϕ – n. E is a constant of motion Two constant of the perturbed motion: μ and C Selecting test particles with fixed μ and C corresponds to look at a certain slice in the phase space (single resonance). - Test particles (macro particles) are evolved in the fields computed in the self-consistent simulation (preprocessing) - collect information on θ=0 plane - phase space structure: mapping on (Pϕ, Θ=nϕ-ωt) X. Wang et al IAEAEP 2015 Vienna 10
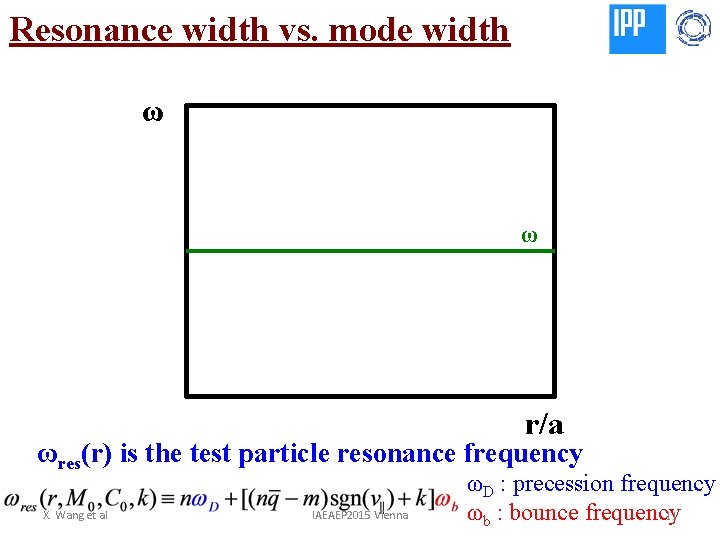
Resonance width vs. mode width ω ω r/a ωres(r) is the test particle resonance frequency X. Wang et al IAEAEP 2015 Vienna ωD : precession frequency ωb : bounce frequency 11
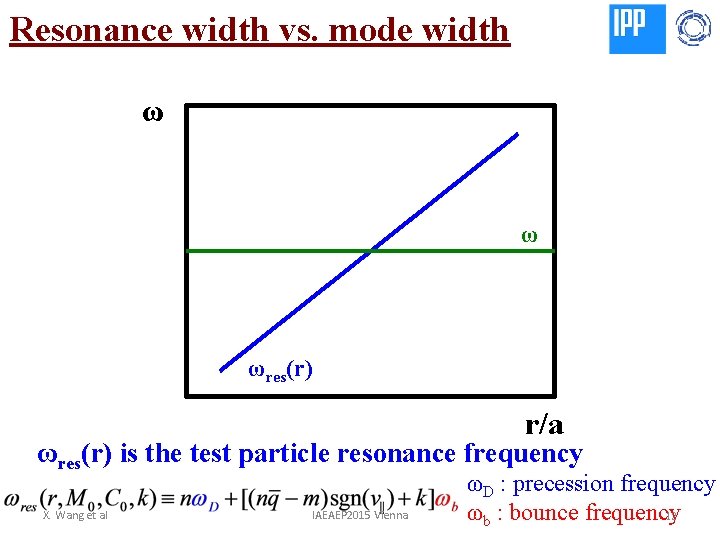
Resonance width vs. mode width ω ω ωres(r) r/a ωres(r) is the test particle resonance frequency X. Wang et al IAEAEP 2015 Vienna ωD : precession frequency ωb : bounce frequency 12
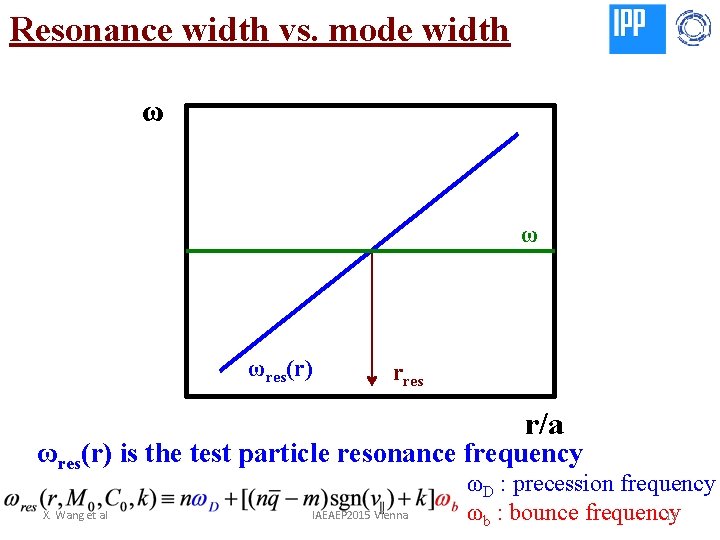
Resonance width vs. mode width ω ω ωres(r) rres r/a ωres(r) is the test particle resonance frequency X. Wang et al IAEAEP 2015 Vienna ωD : precession frequency ωb : bounce frequency 13
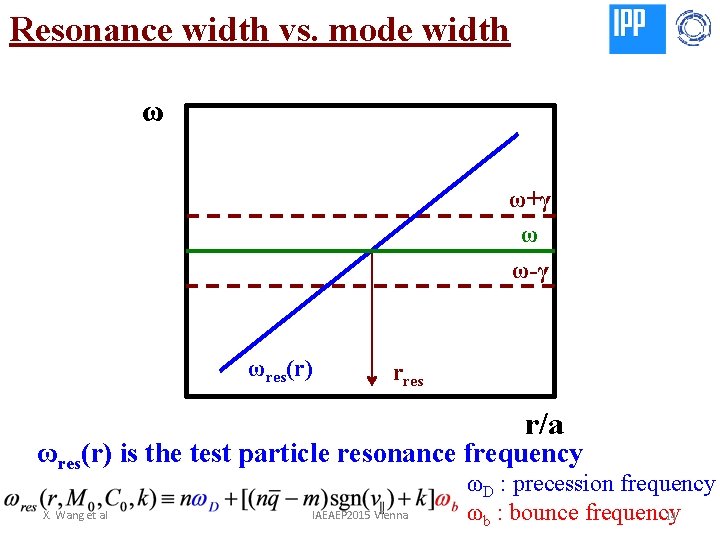
Resonance width vs. mode width ω ω+γ ω ω-γ ωres(r) rres r/a ωres(r) is the test particle resonance frequency X. Wang et al IAEAEP 2015 Vienna ωD : precession frequency ωb : bounce frequency 14
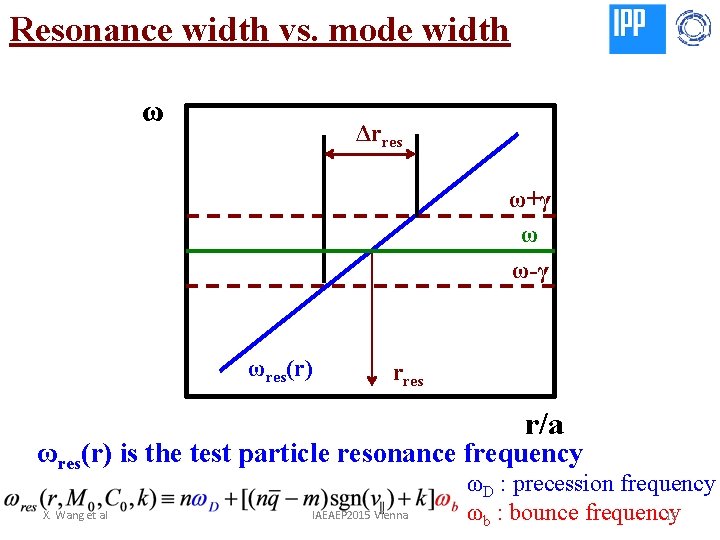
Resonance width vs. mode width ω Δrres ω+γ ω ω-γ ωres(r) rres r/a ωres(r) is the test particle resonance frequency X. Wang et al IAEAEP 2015 Vienna ωD : precession frequency ωb : bounce frequency 15
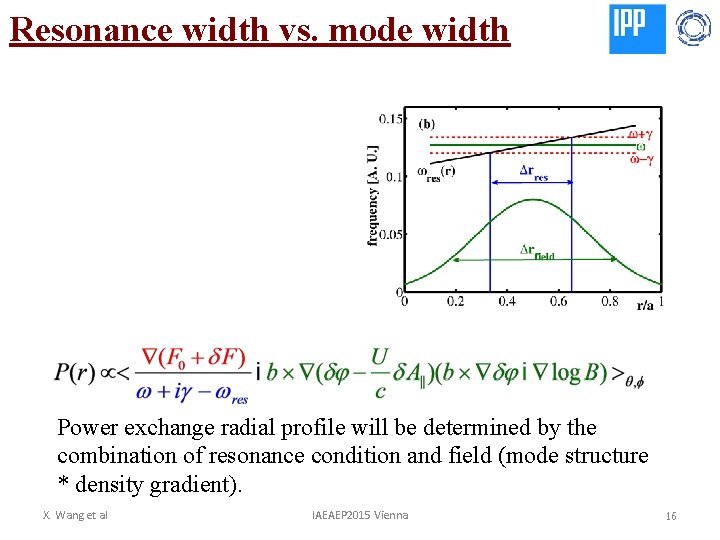
Resonance width vs. mode width Power exchange radial profile will be determined by the combination of resonance condition and field (mode structure * density gradient). X. Wang et al IAEAEP 2015 Vienna 16
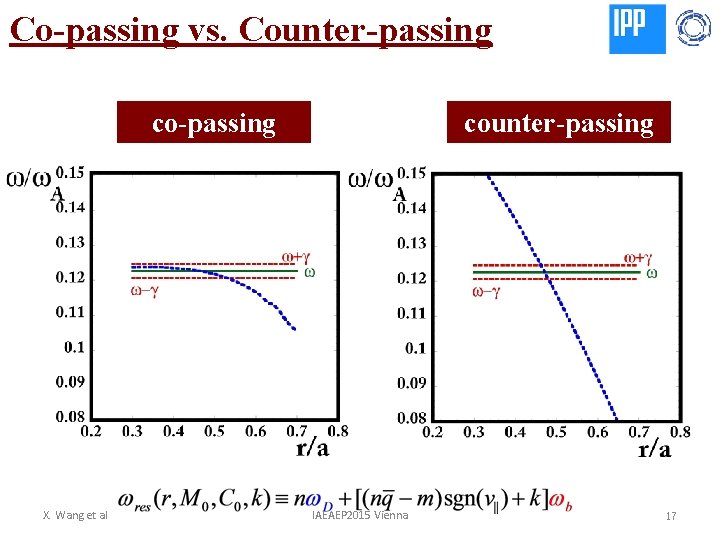
Co-passing vs. Counter-passing co-passing X. Wang et al counter-passing IAEAEP 2015 Vienna 17
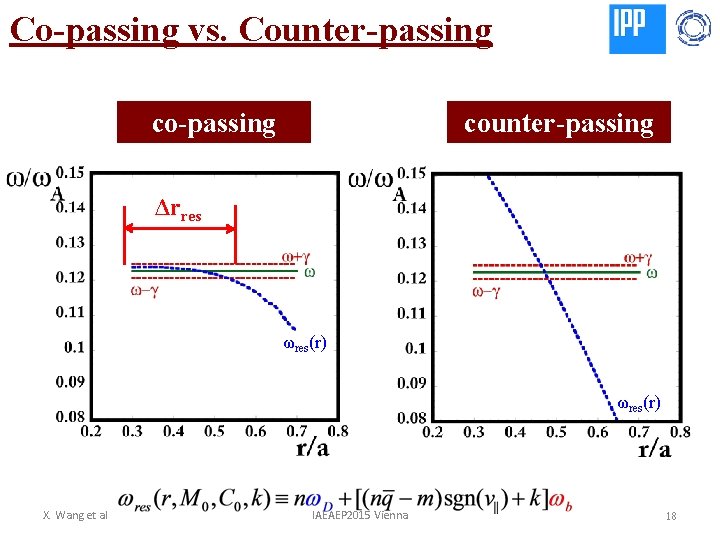
Co-passing vs. Counter-passing counter-passing Δrres ωres(r) X. Wang et al IAEAEP 2015 Vienna 18
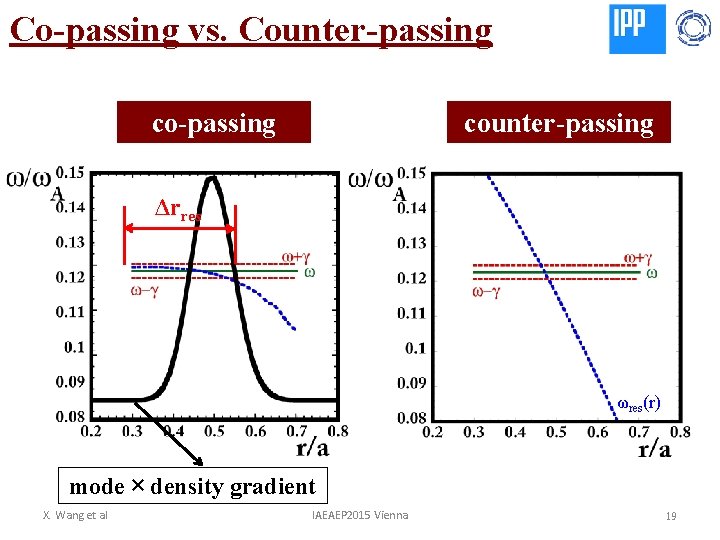
Co-passing vs. Counter-passing counter-passing Δrres ωres(r) mode × density gradient X. Wang et al IAEAEP 2015 Vienna 19
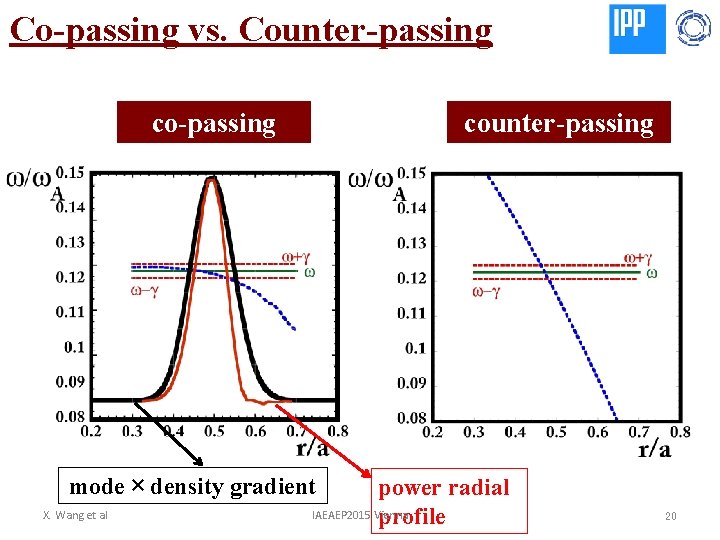
Co-passing vs. Counter-passing counter-passing mode × density gradient X. Wang et al power radial IAEAEP 2015 Vienna profile 20
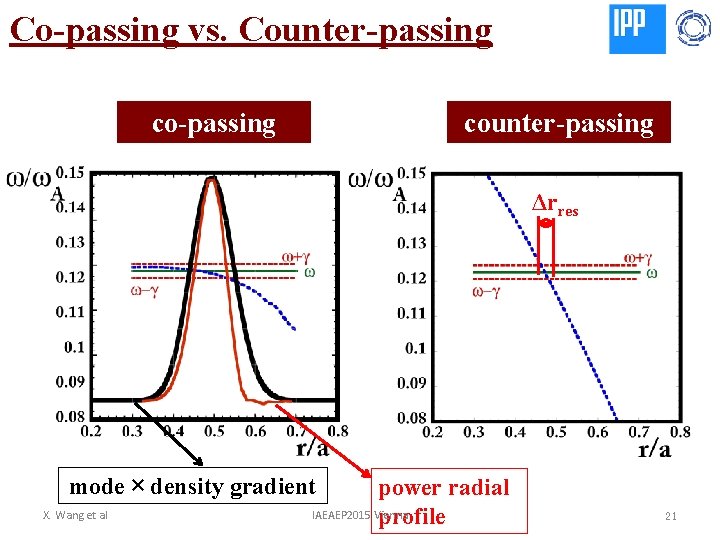
Co-passing vs. Counter-passing counter-passing Δrres mode × density gradient X. Wang et al power radial IAEAEP 2015 Vienna profile 21
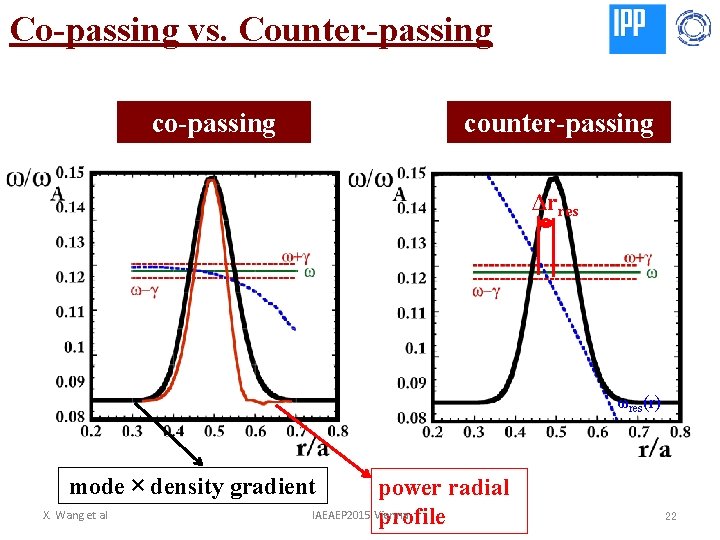
Co-passing vs. Counter-passing counter-passing Δrres ωres(r) mode × density gradient X. Wang et al power radial IAEAEP 2015 Vienna profile 22
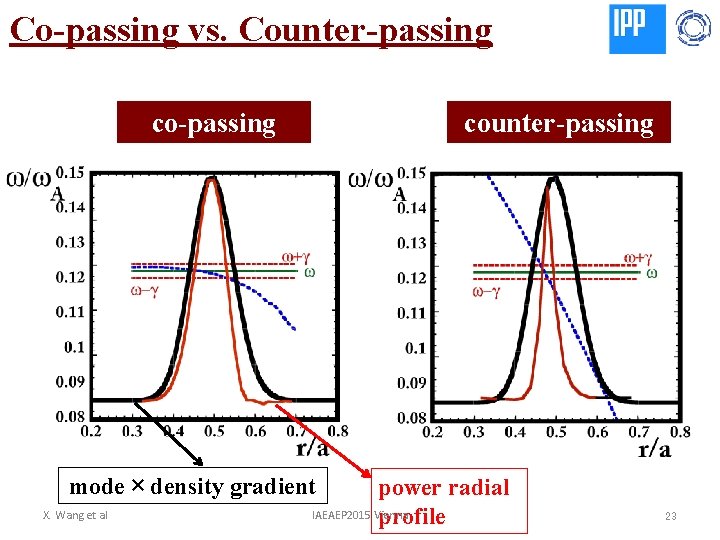
Co-passing vs. Counter-passing counter-passing mode × density gradient X. Wang et al power radial IAEAEP 2015 Vienna profile 23
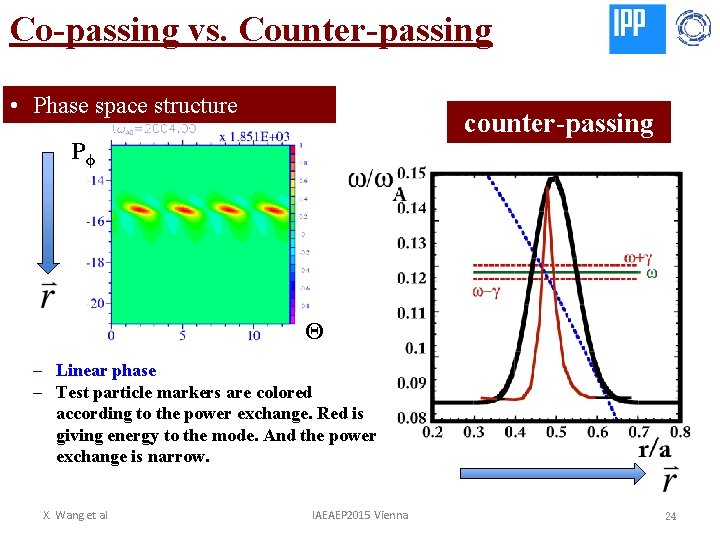
Co-passing vs. Counter-passing • Phase space structure counter-passing Pϕ Θ - Linear phase - Test particle markers are colored according to the power exchange. Red is giving energy to the mode. And the power exchange is narrow. X. Wang et al IAEAEP 2015 Vienna 24
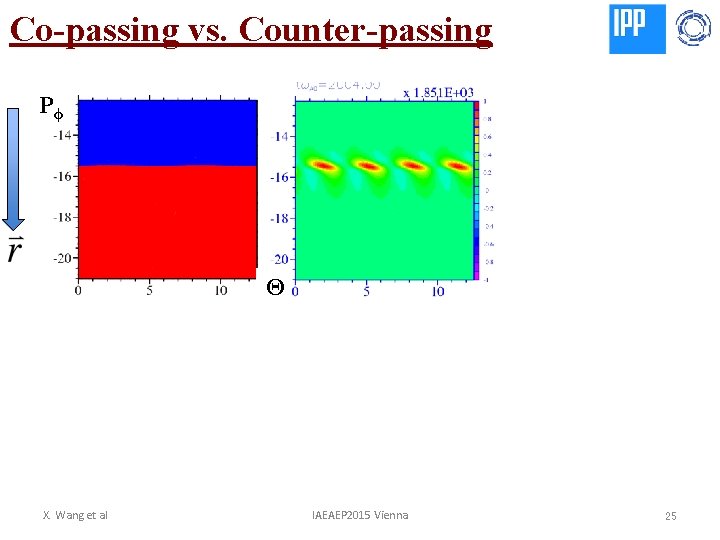
Co-passing vs. Counter-passing Pϕ Θ X. Wang et al IAEAEP 2015 Vienna 25
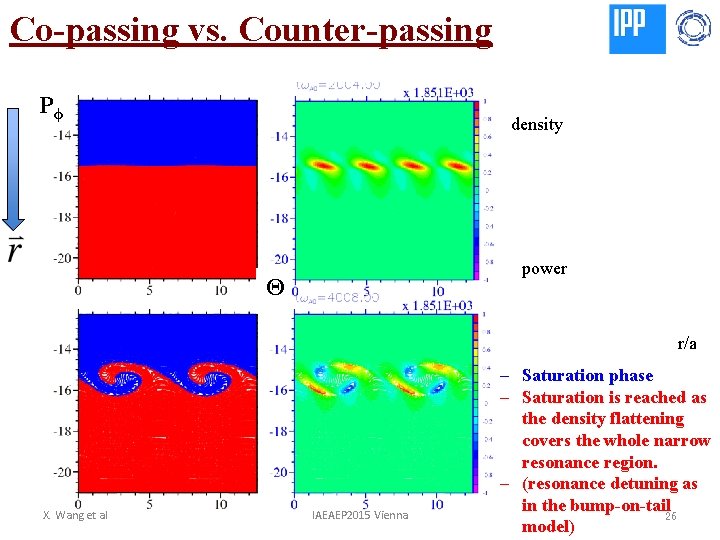
Co-passing vs. Counter-passing Pϕ density power Θ r/a X. Wang et al IAEAEP 2015 Vienna - Saturation phase - Saturation is reached as the density flattening covers the whole narrow resonance region. - (resonance detuning as in the bump-on-tail 26 model)
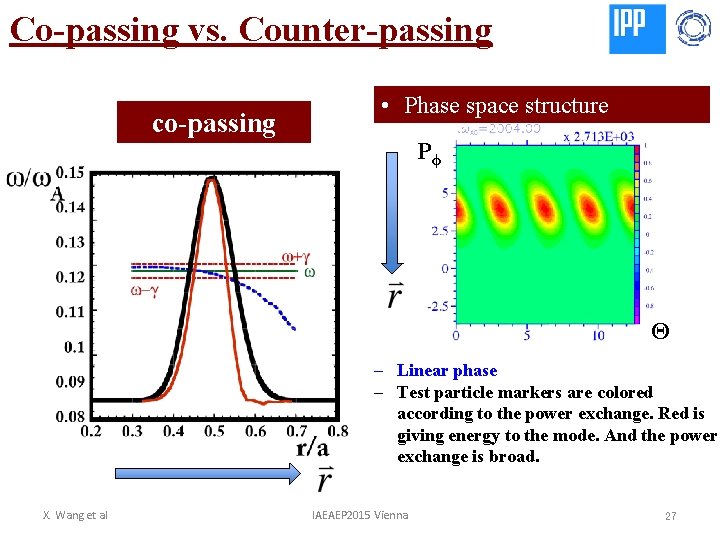
Co-passing vs. Counter-passing co-passing • Phase space structure Pϕ Θ - Linear phase - Test particle markers are colored according to the power exchange. Red is giving energy to the mode. And the power exchange is broad. X. Wang et al IAEAEP 2015 Vienna 27
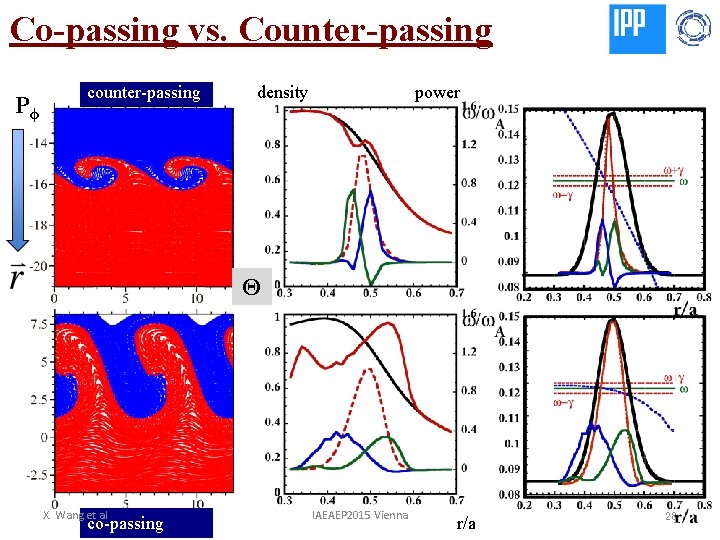
Co-passing vs. Counter-passing Pϕ counter-passing density power Θ X. Wang et al co-passing IAEAEP 2015 Vienna r/a 28
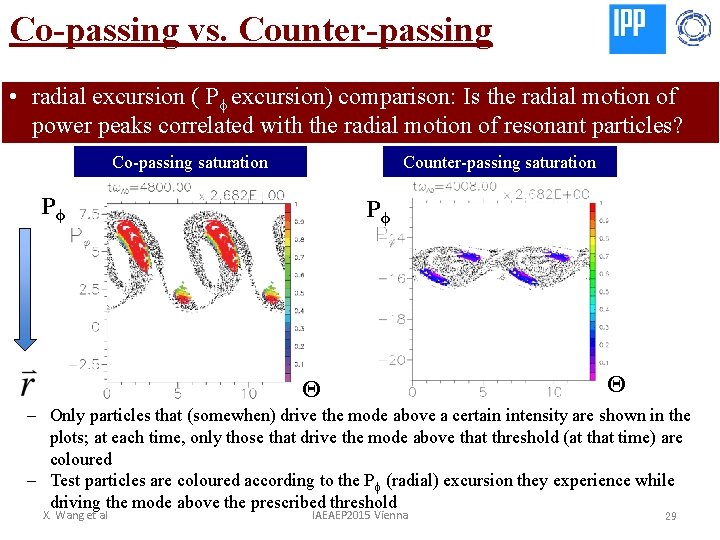
Co-passing vs. Counter-passing • radial excursion ( Pϕ excursion) comparison: Is the radial motion of power peaks correlated with the radial motion of resonant particles? Co-passing saturation Counter-passing saturation Pϕ Pϕ Θ Θ - Only particles that (somewhen) drive the mode above a certain intensity are shown in the plots; at each time, only those that drive the mode above that threshold (at that time) are coloured - Test particles are coloured according to the Pϕ (radial) excursion they experience while driving the mode above the prescribed threshold X. Wang et al IAEAEP 2015 Vienna 29
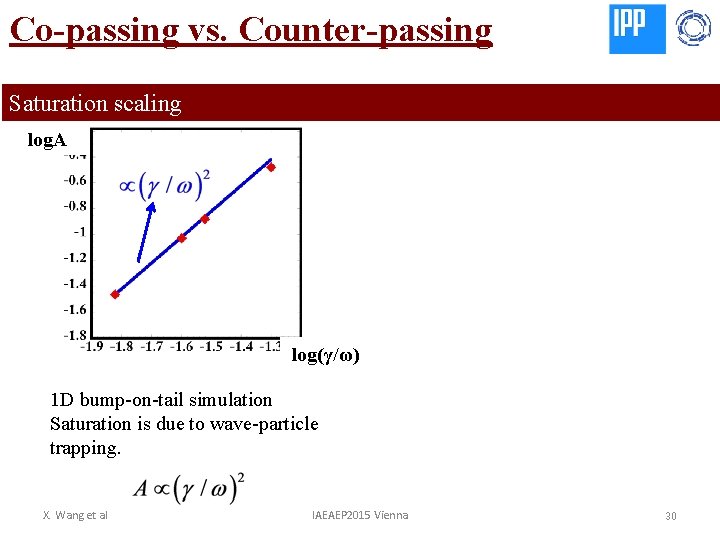
Co-passing vs. Counter-passing Saturation scaling log. A log(γ/ω) 1 D bump-on-tail simulation Saturation is due to wave-particle trapping. X. Wang et al IAEAEP 2015 Vienna 30
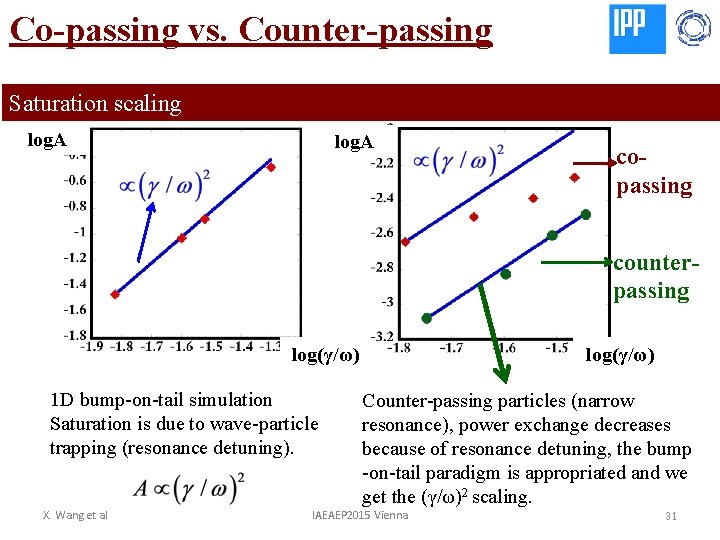
Co-passing vs. Counter-passing Saturation scaling log. A copassing counterpassing log(γ/ω) 1 D bump-on-tail simulation Saturation is due to wave-particle trapping (resonance detuning). X. Wang et al log(γ/ω) Counter-passing particles (narrow resonance), power exchange decreases because of resonance detuning, the bump -on-tail paradigm is appropriated and we get the (γ/ω)2 scaling. IAEAEP 2015 Vienna 31
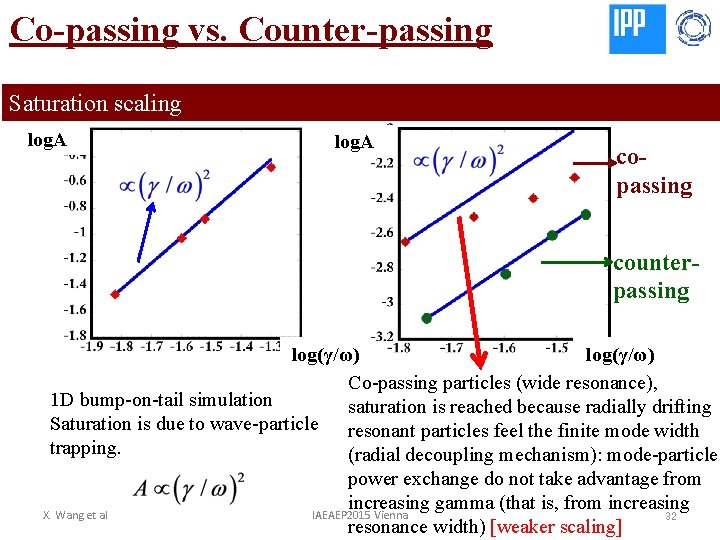
Co-passing vs. Counter-passing Saturation scaling log. A copassing counterpassing log(γ/ω) Co-passing particles (wide resonance), 1 D bump-on-tail simulation saturation is reached because radially drifting Saturation is due to wave-particle resonant particles feel the finite mode width trapping. (radial decoupling mechanism): mode-particle power exchange do not take advantage from increasing gamma (that is, from increasing X. Wang et al IAEAEP 2015 Vienna 32 resonance width) [weaker scaling]
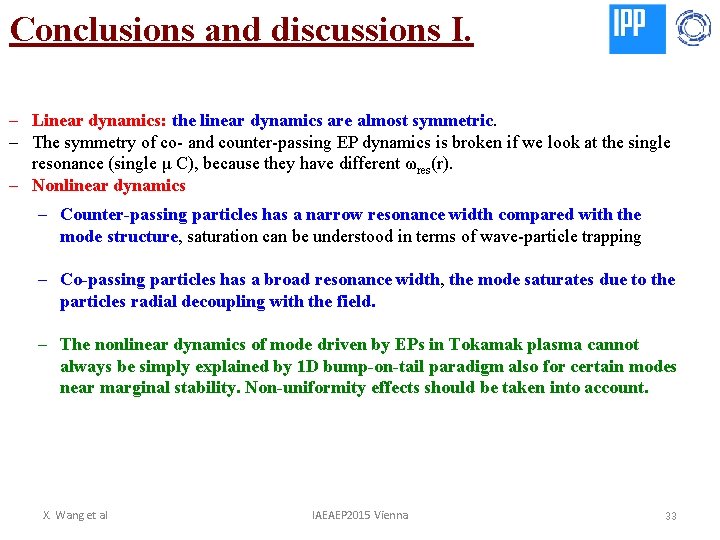
Conclusions and discussions I. - Linear dynamics: the linear dynamics are almost symmetric. - The symmetry of co- and counter-passing EP dynamics is broken if we look at the single resonance (single μ C), because they have different ωres(r). - Nonlinear dynamics - Counter-passing particles has a narrow resonance width compared with the mode structure, saturation can be understood in terms of wave-particle trapping - Co-passing particles has a broad resonance width, the mode saturates due to the particles radial decoupling with the field. - The nonlinear dynamics of mode driven by EPs in Tokamak plasma cannot always be simply explained by 1 D bump-on-tail paradigm also for certain modes near marginal stability. Non-uniformity effects should be taken into account. X. Wang et al IAEAEP 2015 Vienna 33
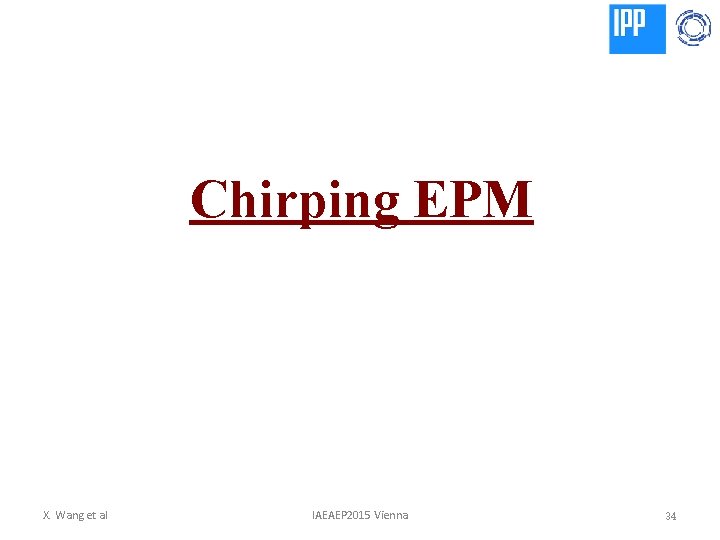
Chirping EPM X. Wang et al IAEAEP 2015 Vienna 34
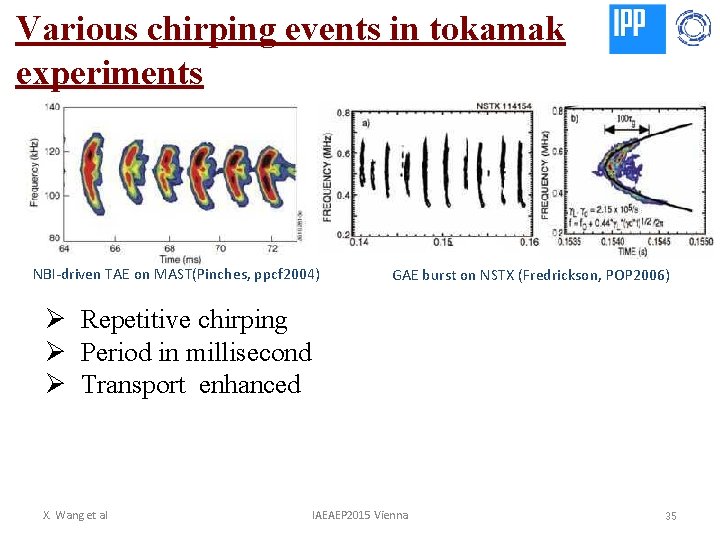
Various chirping events in tokamak experiments NBI-driven TAE on MAST(Pinches, ppcf 2004) GAE burst on NSTX (Fredrickson, POP 2006) Ø Repetitive chirping Ø Period in millisecond Ø Transport enhanced X. Wang et al IAEAEP 2015 Vienna 35
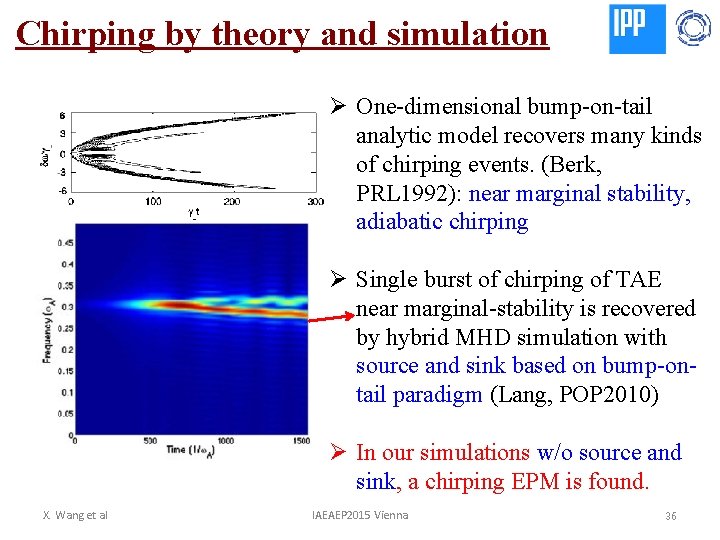
Chirping by theory and simulation Ø One-dimensional bump-on-tail analytic model recovers many kinds of chirping events. (Berk, PRL 1992): near marginal stability, adiabatic chirping Ø Single burst of chirping of TAE near marginal-stability is recovered by hybrid MHD simulation with source and sink based on bump-ontail paradigm (Lang, POP 2010) Ø In our simulations w/o source and sink, a chirping EPM is found. X. Wang et al IAEAEP 2015 Vienna 36
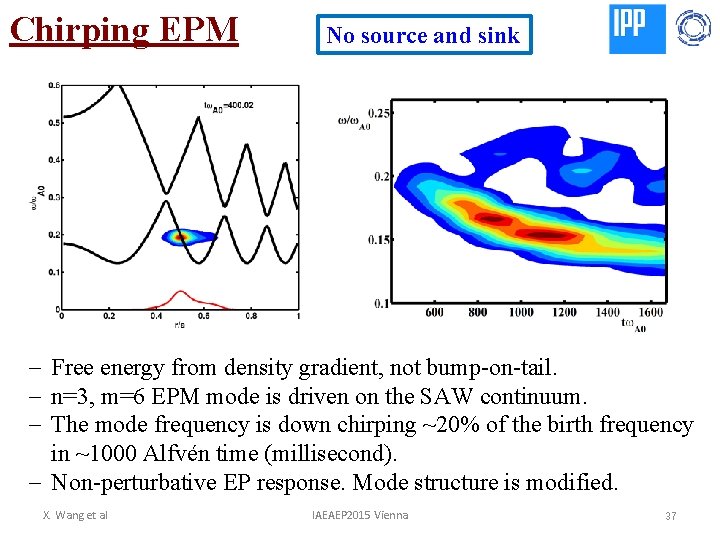
Chirping EPM No source and sink - Free energy from density gradient, not bump-on-tail. - n=3, m=6 EPM mode is driven on the SAW continuum. - The mode frequency is down chirping ~20% of the birth frequency in ~1000 Alfvén time (millisecond). - Non-perturbative EP response. Mode structure is modified. X. Wang et al IAEAEP 2015 Vienna 37
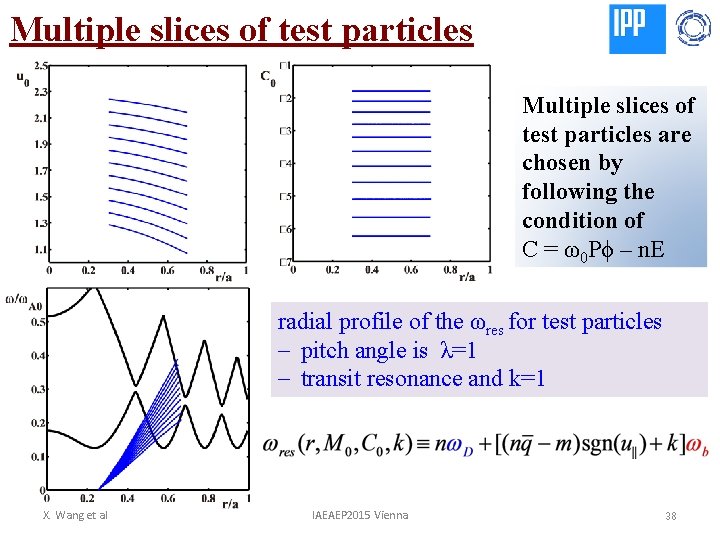
Multiple slices of test particles are chosen by following the condition of C = ω0 Pϕ – n. E radial profile of the ωres for test particles - pitch angle is λ=1 - transit resonance and k=1 X. Wang et al IAEAEP 2015 Vienna 38
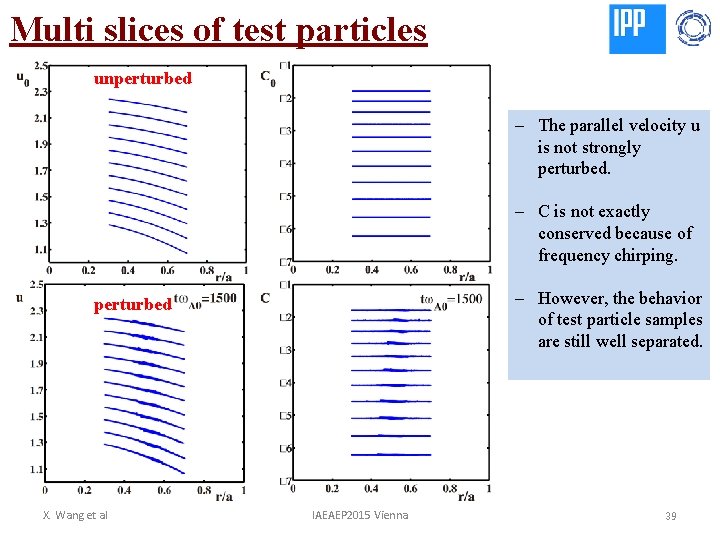
Multi slices of test particles unperturbed - The parallel velocity u is not strongly perturbed. - C is not exactly conserved because of frequency chirping. - However, the behavior of test particle samples are still well separated. perturbed X. Wang et al IAEAEP 2015 Vienna 39
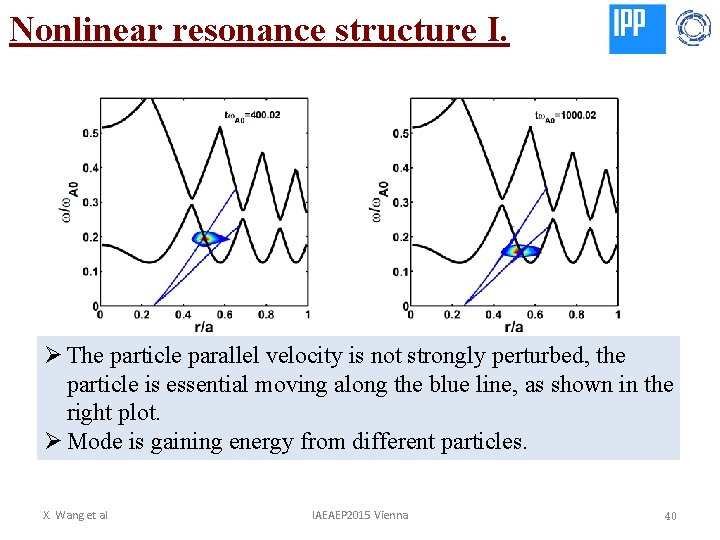
Nonlinear resonance structure I. Ø The particle parallel velocity is not strongly perturbed, the particle is essential moving along the blue line, as shown in the right plot. Ø Mode is gaining energy from different particles. X. Wang et al IAEAEP 2015 Vienna 40
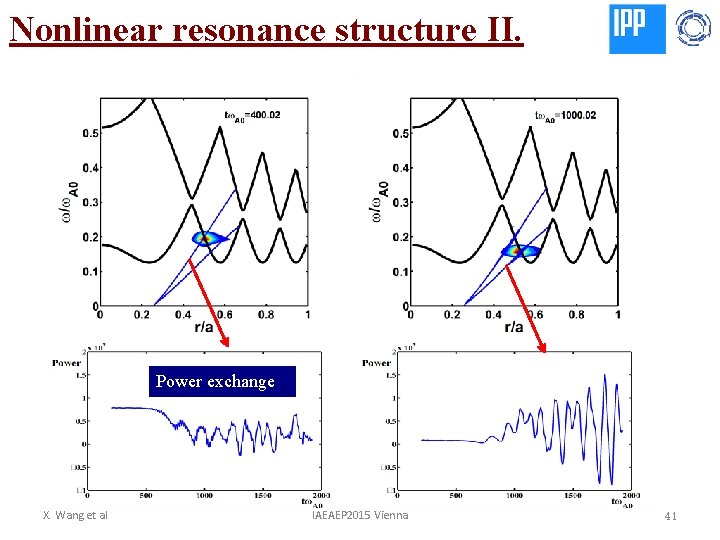
Nonlinear resonance structure II. Power exchange X. Wang et al IAEAEP 2015 Vienna 41
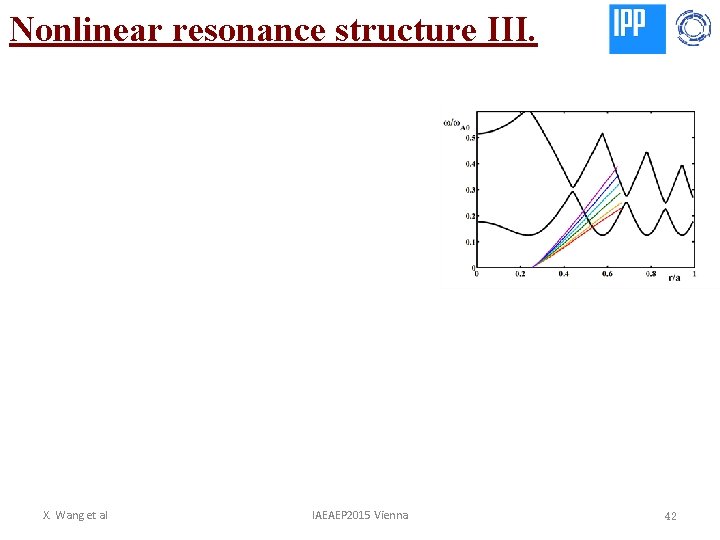
Nonlinear resonance structure III. X. Wang et al IAEAEP 2015 Vienna 42
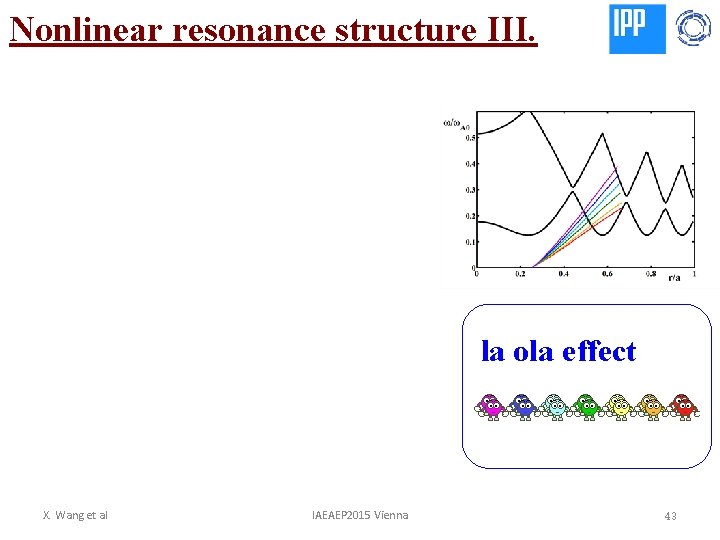
Nonlinear resonance structure III. la ola effect X. Wang et al IAEAEP 2015 Vienna 43
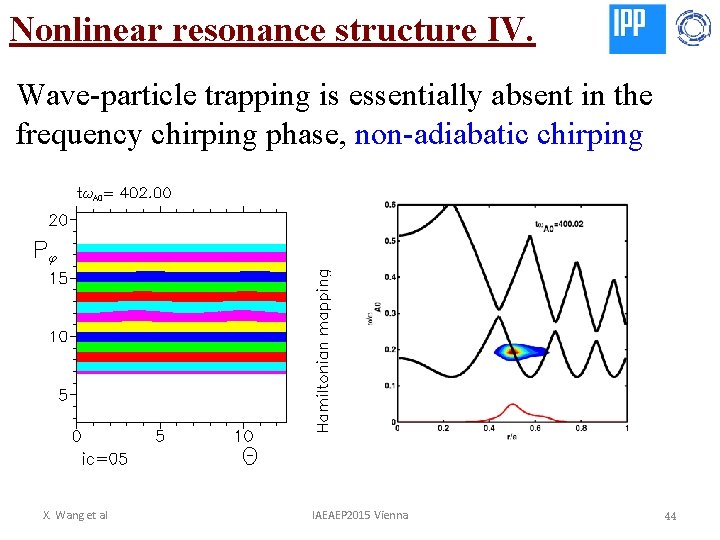
Nonlinear resonance structure IV. Wave-particle trapping is essentially absent in the frequency chirping phase, non-adiabatic chirping X. Wang et al IAEAEP 2015 Vienna 44
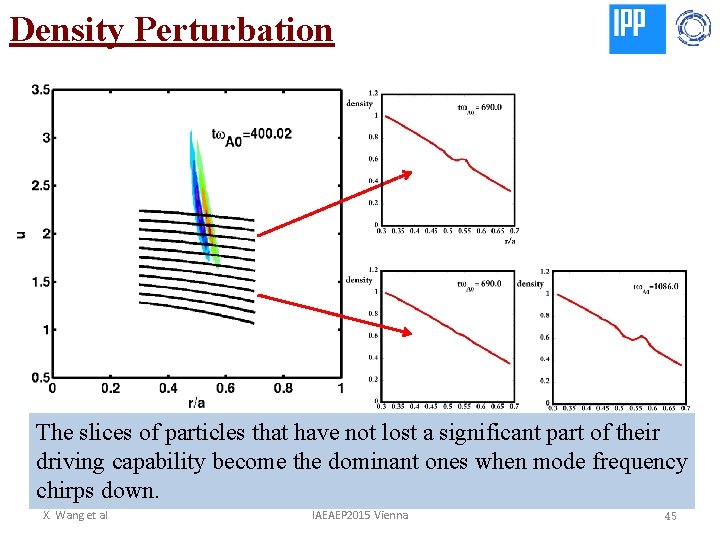
Density Perturbation The slices of particles that have not lost a significant part of their driving capability become the dominant ones when mode frequency chirps down. X. Wang et al IAEAEP 2015 Vienna 45
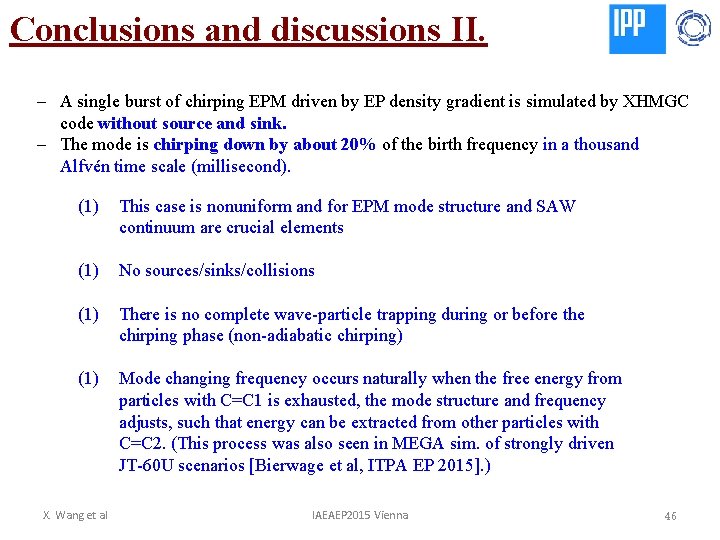
Conclusions and discussions II. - A single burst of chirping EPM driven by EP density gradient is simulated by XHMGC code without source and sink. - The mode is chirping down by about 20% of the birth frequency in a thousand Alfvén time scale (millisecond). (1) This case is nonuniform and for EPM mode structure and SAW continuum are crucial elements (1) No sources/sinks/collisions (1) There is no complete wave-particle trapping during or before the chirping phase (non-adiabatic chirping) (1) Mode changing frequency occurs naturally when the free energy from particles with C=C 1 is exhausted, the mode structure and frequency adjusts, such that energy can be extracted from other particles with C=C 2. (This process was also seen in MEGA sim. of strongly driven JT-60 U scenarios [Bierwage et al, ITPA EP 2015]. ) X. Wang et al IAEAEP 2015 Vienna 46
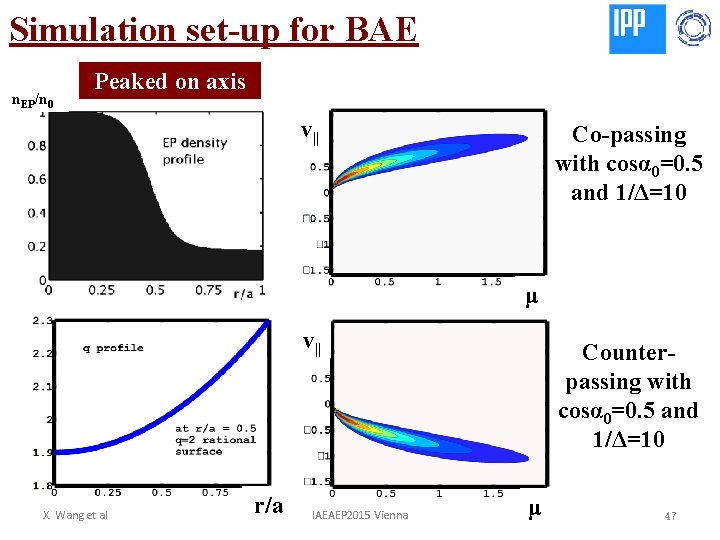
Simulation set-up for BAE n. EP/n 0 Peaked on axis v|| Co-passing with cosα 0=0. 5 and 1/Δ=10 μ v|| X. Wang et al r/a IAEAEP 2015 Vienna Counterpassing with cosα 0=0. 5 and 1/Δ=10 μ 47
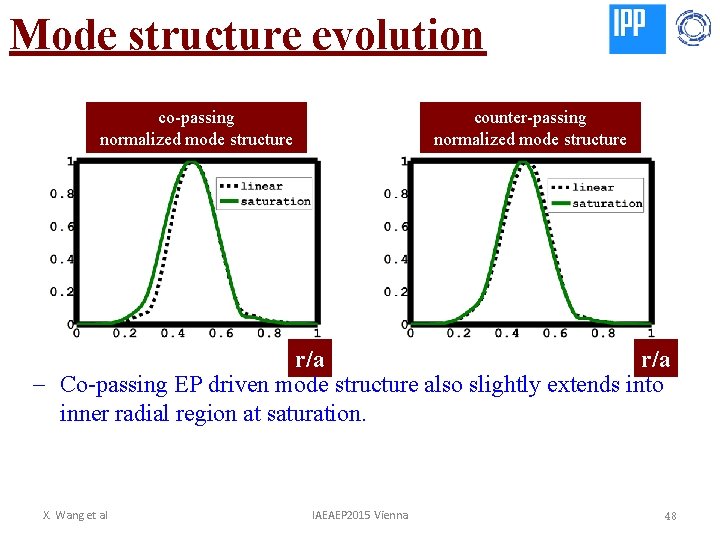
Mode structure evolution co-passing normalized mode structure counter-passing normalized mode structure r/a - Co-passing EP driven mode structure also slightly extends into inner radial region at saturation. X. Wang et al IAEAEP 2015 Vienna 48
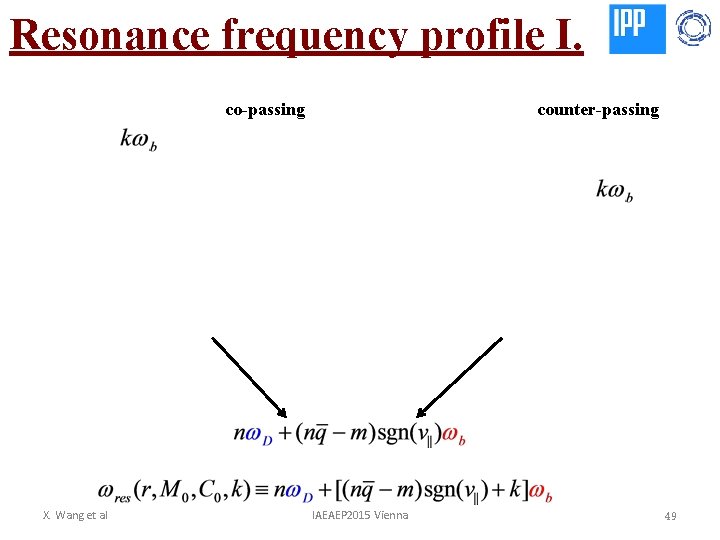
Resonance frequency profile I. co-passing X. Wang et al counter-passing IAEAEP 2015 Vienna 49
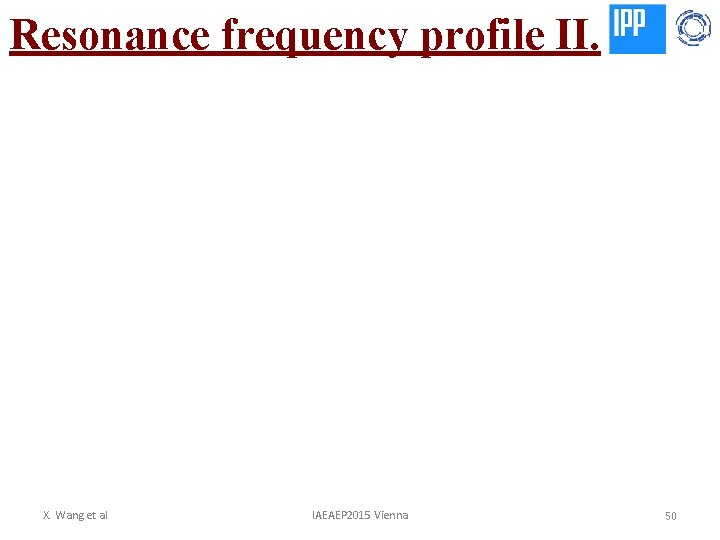
Resonance frequency profile II. X. Wang et al IAEAEP 2015 Vienna 50
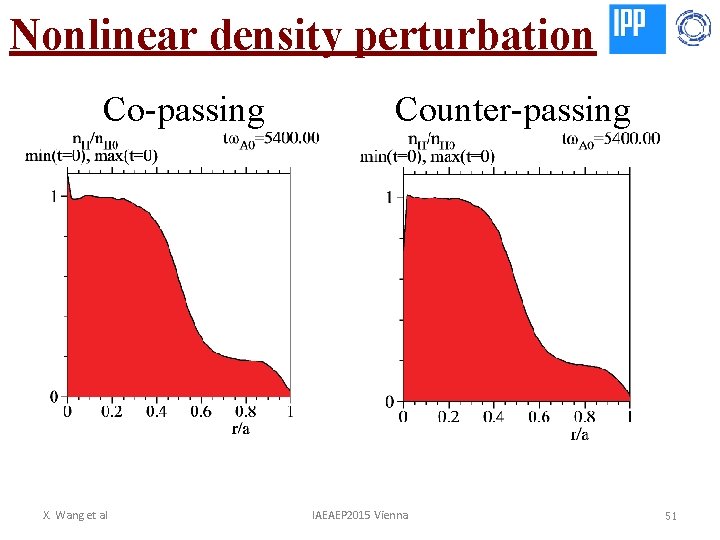
Nonlinear density perturbation Co-passing X. Wang et al Counter-passing IAEAEP 2015 Vienna 51
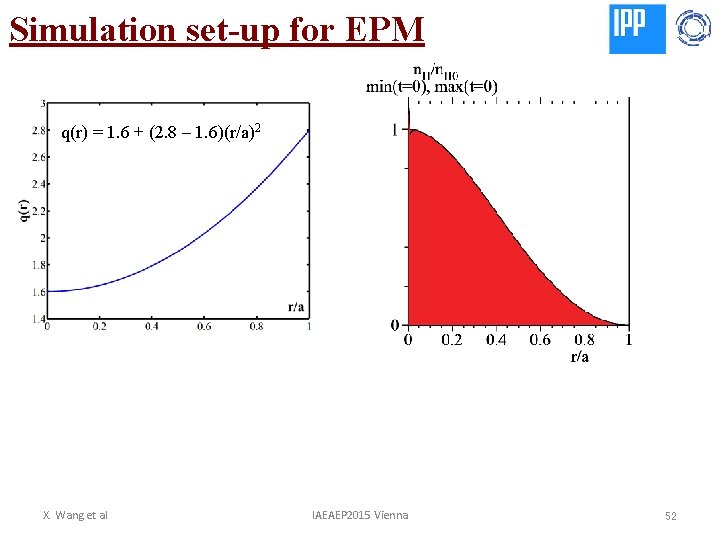
Simulation set-up for EPM q(r) = 1. 6 + (2. 8 – 1. 6)(r/a)2 X. Wang et al IAEAEP 2015 Vienna 52
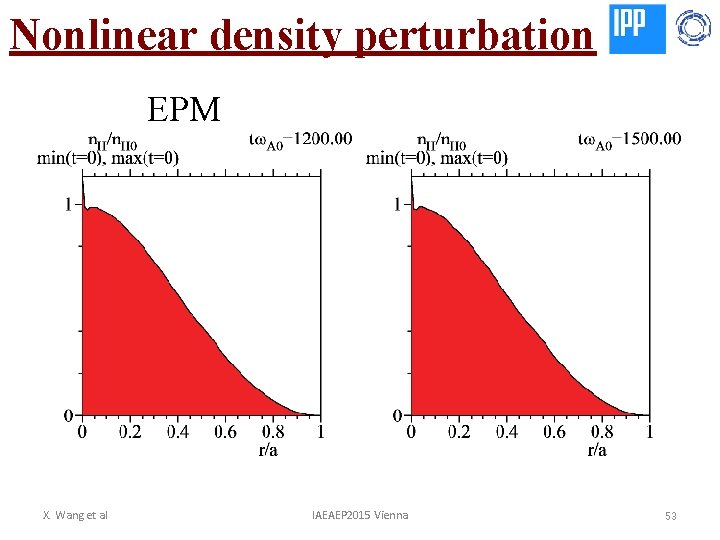
Nonlinear density perturbation EPM X. Wang et al IAEAEP 2015 Vienna 53
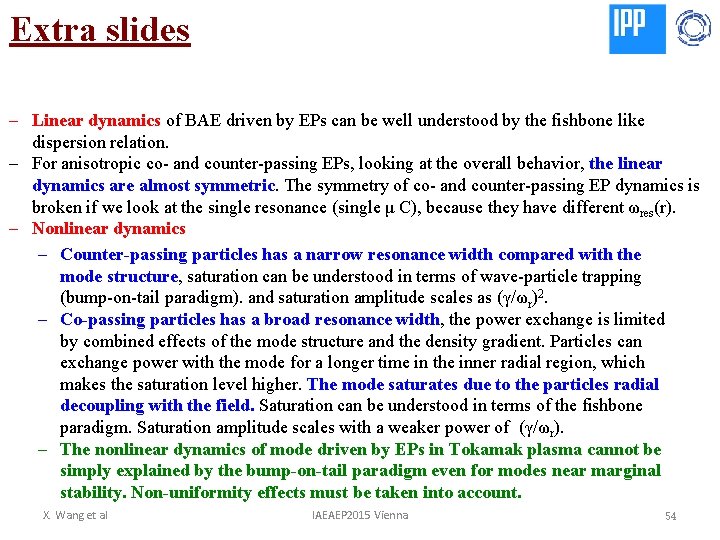
Extra slides - Linear dynamics of BAE driven by EPs can be well understood by the fishbone like dispersion relation. - For anisotropic co- and counter-passing EPs, looking at the overall behavior, the linear dynamics are almost symmetric. The symmetry of co- and counter-passing EP dynamics is broken if we look at the single resonance (single μ C), because they have different ωres(r). - Nonlinear dynamics - Counter-passing particles has a narrow resonance width compared with the mode structure, saturation can be understood in terms of wave-particle trapping (bump-on-tail paradigm). and saturation amplitude scales as (γ/ωr)2. - Co-passing particles has a broad resonance width, the power exchange is limited by combined effects of the mode structure and the density gradient. Particles can exchange power with the mode for a longer time in the inner radial region, which makes the saturation level higher. The mode saturates due to the particles radial decoupling with the field. Saturation can be understood in terms of the fishbone paradigm. Saturation amplitude scales with a weaker power of (γ/ωr). - The nonlinear dynamics of mode driven by EPs in Tokamak plasma cannot be simply explained by the bump-on-tail paradigm even for modes near marginal stability. Non-uniformity effects must be taken into account. X. Wang et al IAEAEP 2015 Vienna 54
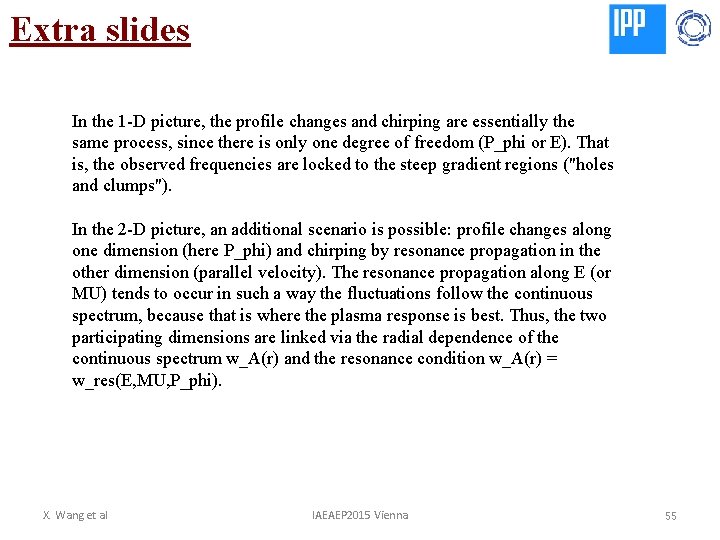
Extra slides In the 1 -D picture, the profile changes and chirping are essentially the same process, since there is only one degree of freedom (P_phi or E). That is, the observed frequencies are locked to the steep gradient regions ("holes and clumps"). In the 2 -D picture, an additional scenario is possible: profile changes along one dimension (here P_phi) and chirping by resonance propagation in the other dimension (parallel velocity). The resonance propagation along E (or MU) tends to occur in such a way the fluctuations follow the continuous spectrum, because that is where the plasma response is best. Thus, the two participating dimensions are linked via the radial dependence of the continuous spectrum w_A(r) and the resonance condition w_A(r) = w_res(E, MU, P_phi). X. Wang et al IAEAEP 2015 Vienna 55
Contoh random fluctuation
Cross fluctuation test in swelling
What is flux analysis in accounting
Contoh fluctuation stock
Intervalle de fluctuation
Régime de change au maroc
Ebullience synonym
Linear and nonlinear data structure
Linear and nonlinear data structure
Nonlinear plot examples
Noncovalent interactions
Congress formal and informal powers
Competition symbiotic relationship
Wikimedia
Qualitative and quantitative research
Sertraline interactions
Macroeconomics unit 5 lesson 2 activity 45
The properties and interactions of magnets
Grapefruit-drug interactions chart
Interactions in the environment grade 7
Biotic
What are sphere interactions
Section 20-1 review species interactions
Flocabulary photosynthesis read and respond answers
Habitats niches and species interactions
Moa of h2 antagonist
Types of interactions
Circulatory system interactions with other systems
Modular vs integral product architecture
Axial substituents
Diazepam cyp450
5 factors of effective communication
Symbiosis and species interactions keystone webquest
Circulatory system interactions with other systems
Naive bayes pays attention to complex interactions and
Chapter 22 reaching out cross-cultural interactions
Vitamin e main function
Modular vs integral product architecture
Special interactions
Cholinomimetic
Chapter 22 reaching out cross-cultural interactions
Chapter 22 reaching out cross-cultural interactions
Accounting information system chapter 1
Which of the following tells you population density
Heterotrophs
Protein binding interactions
Nutrient interactions
Foss chemical interactions
Niches and community interactions
Interactions
Synergism
Ecosystems interactions
Intramolecular interactions
Protein binding interactions
Intramolecular forces
Protein binding interactions
Wave interactions