Electrochemistry MAE212 Dr Marc Madou UCI Winter 2017
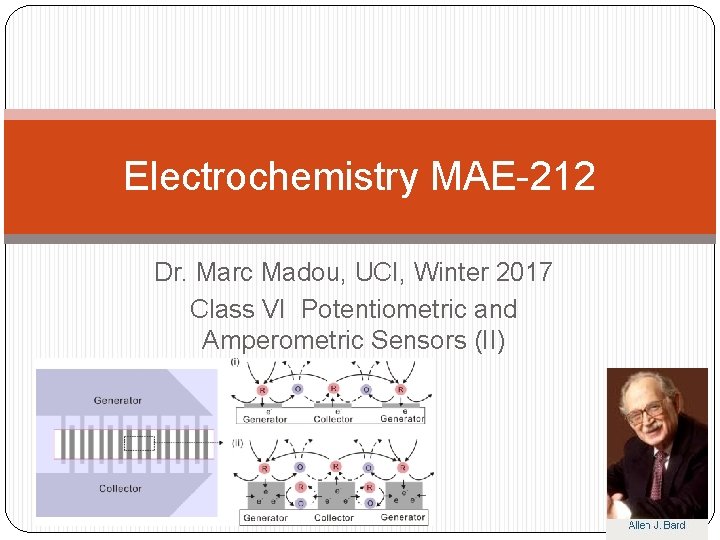
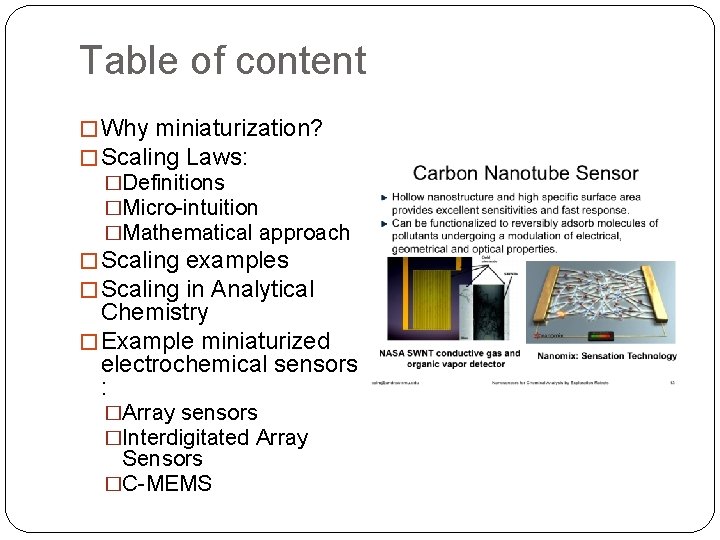
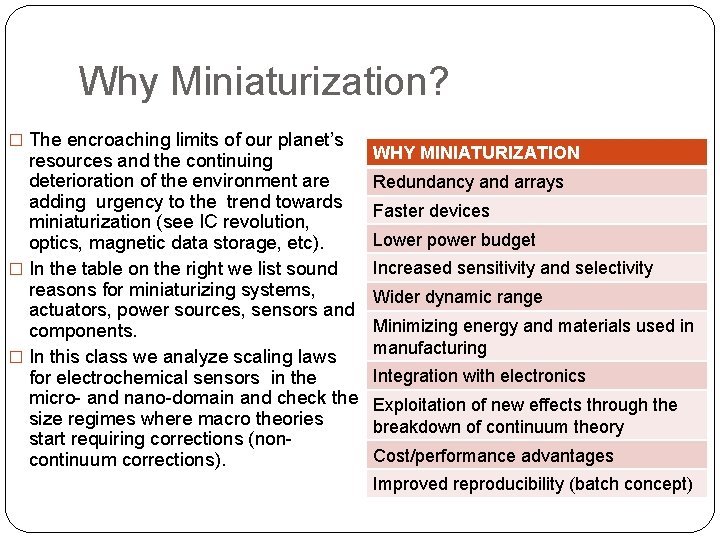
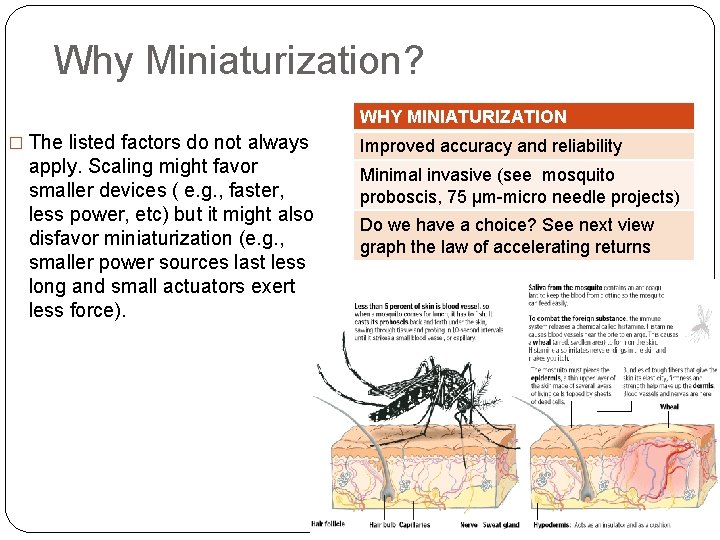
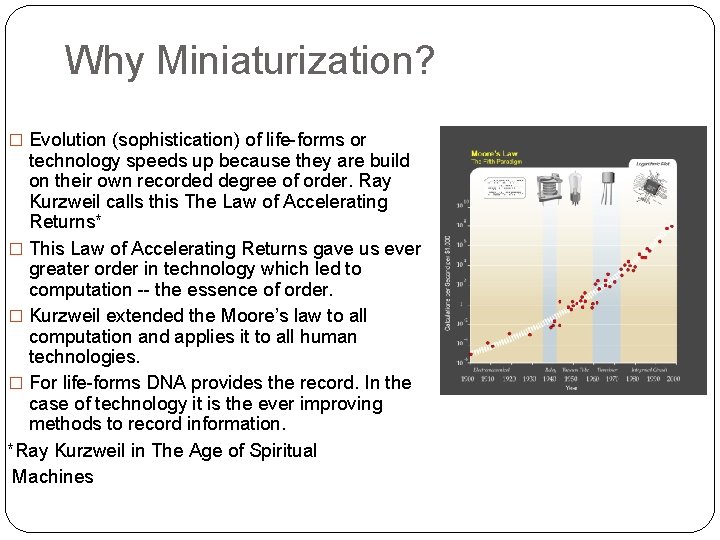
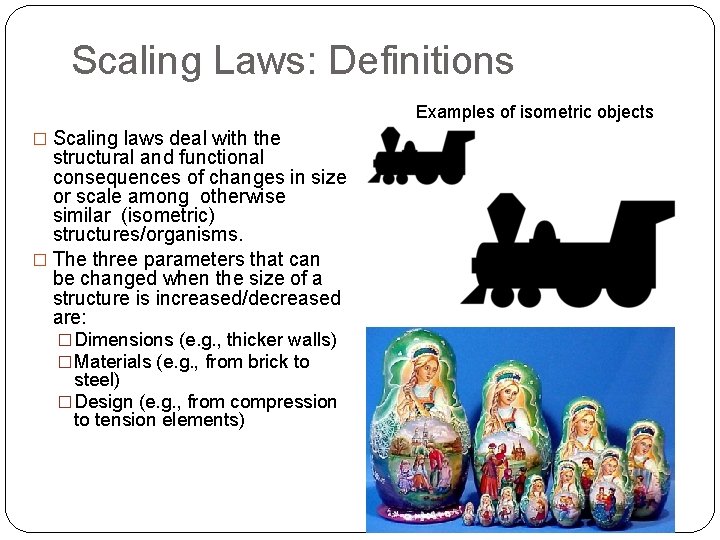
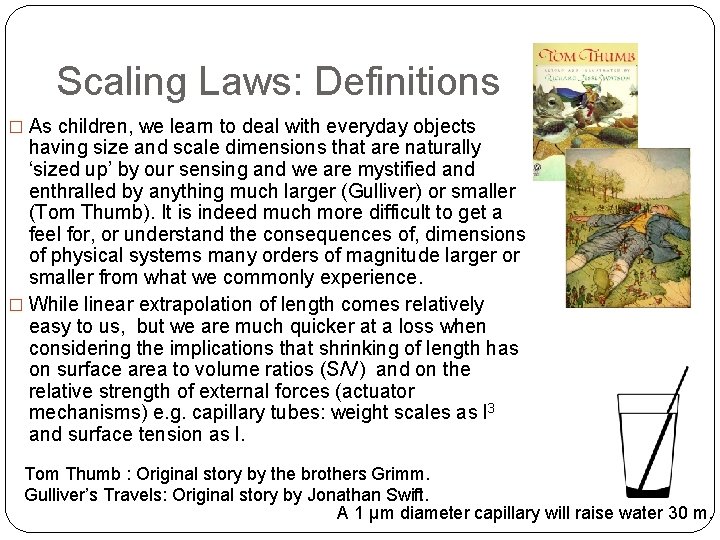
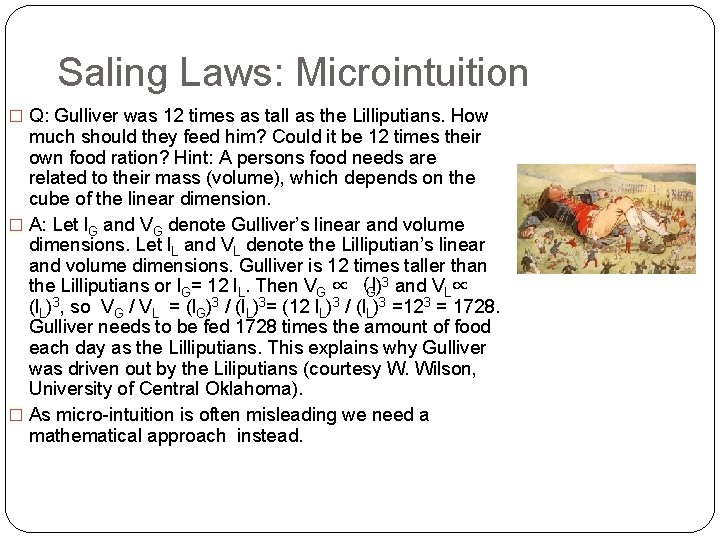
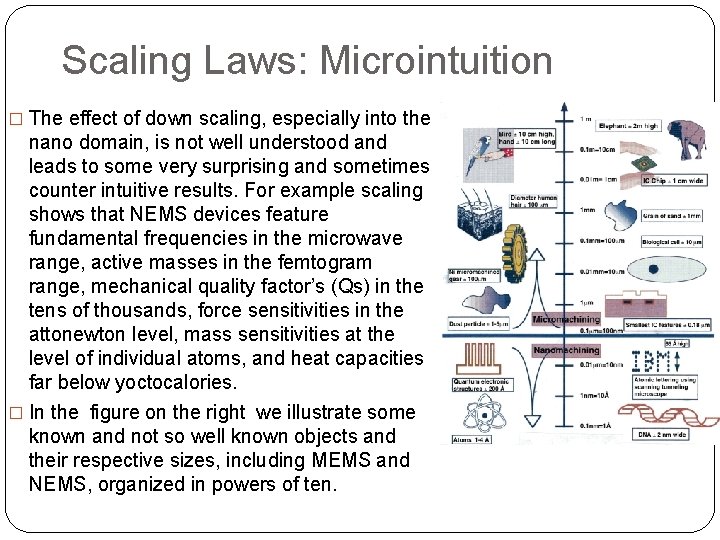
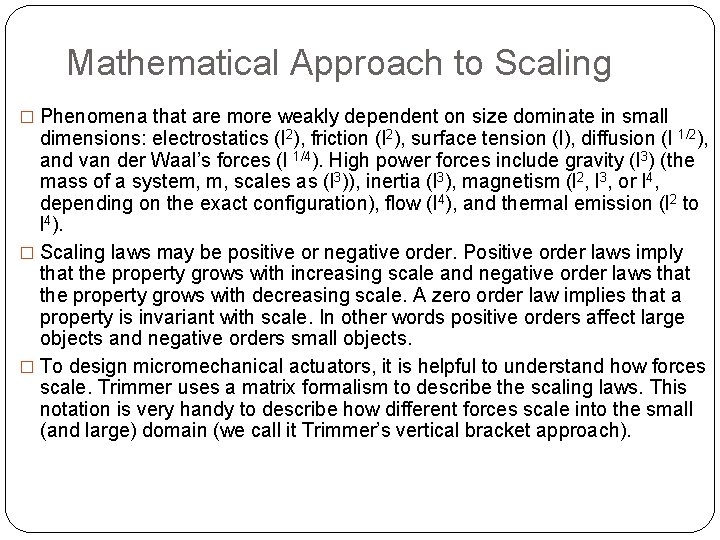
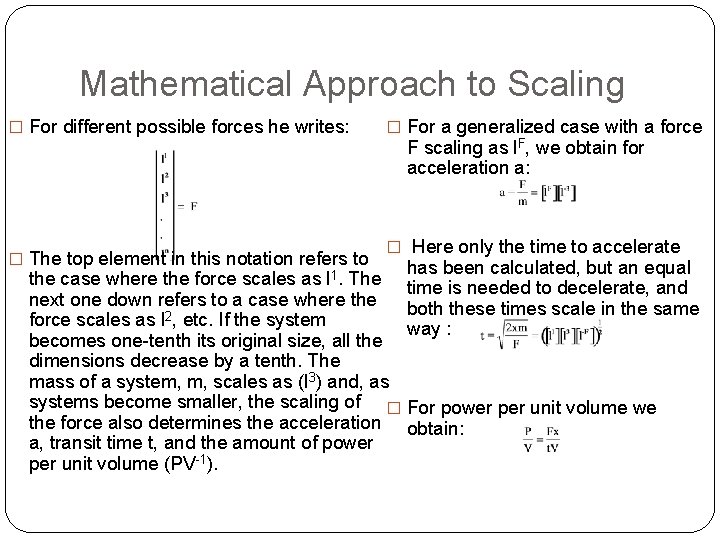
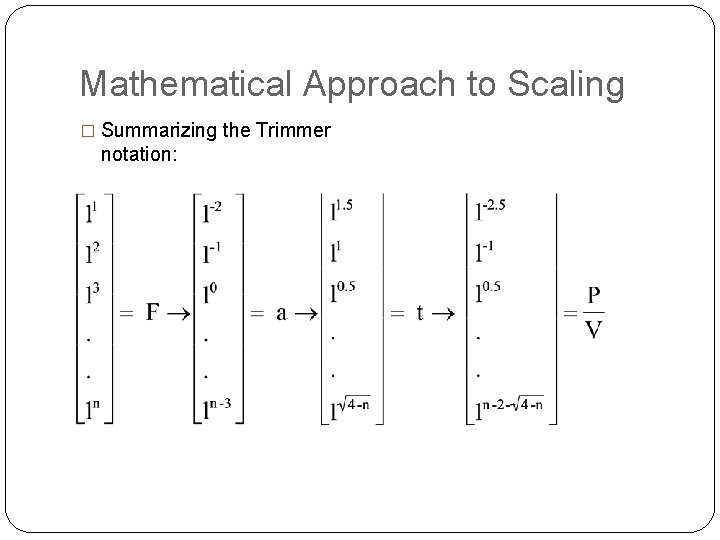
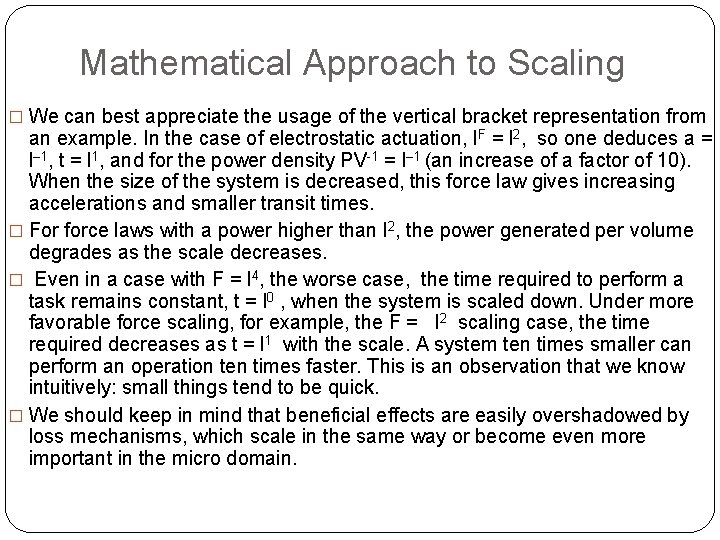
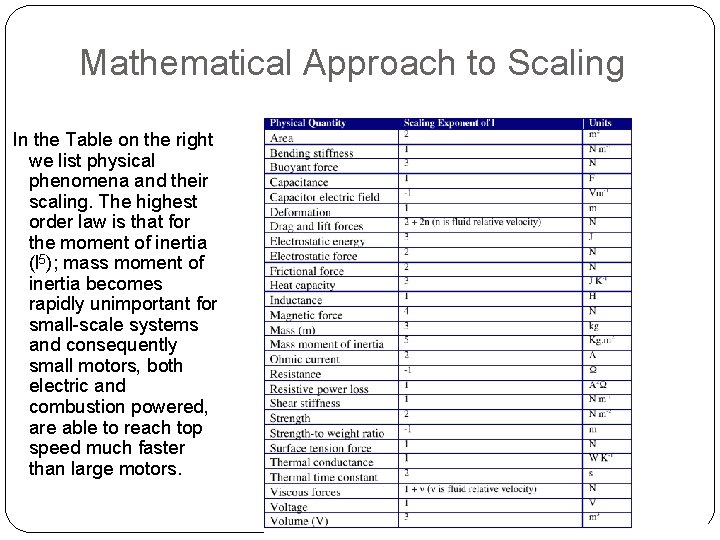
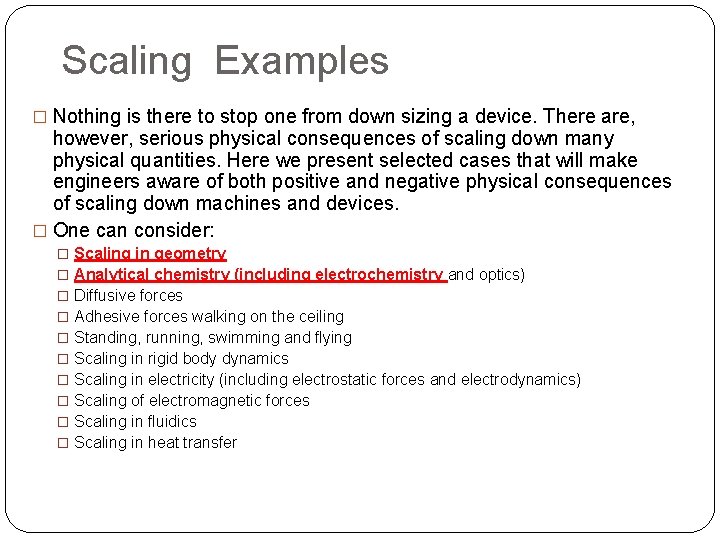
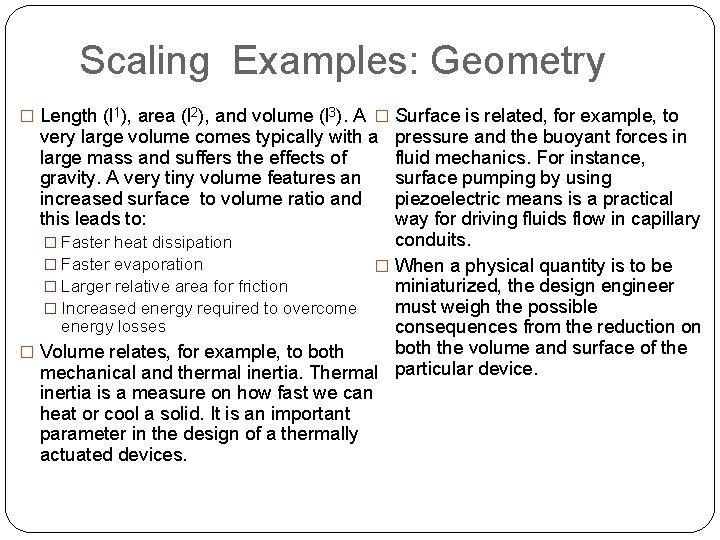
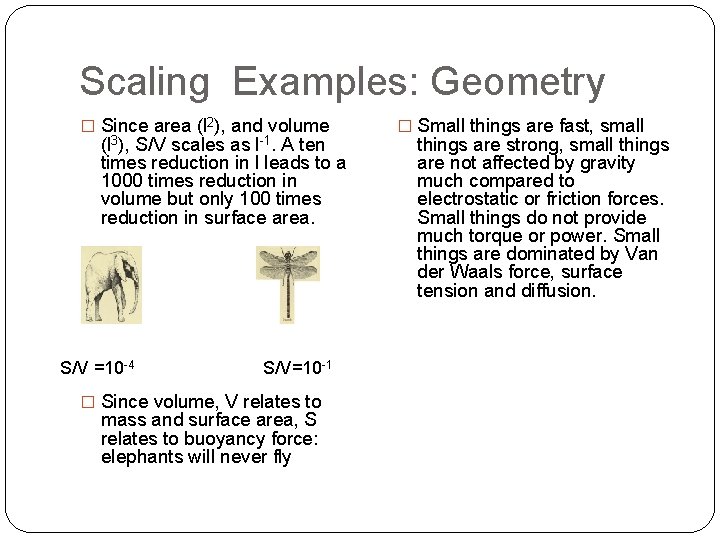
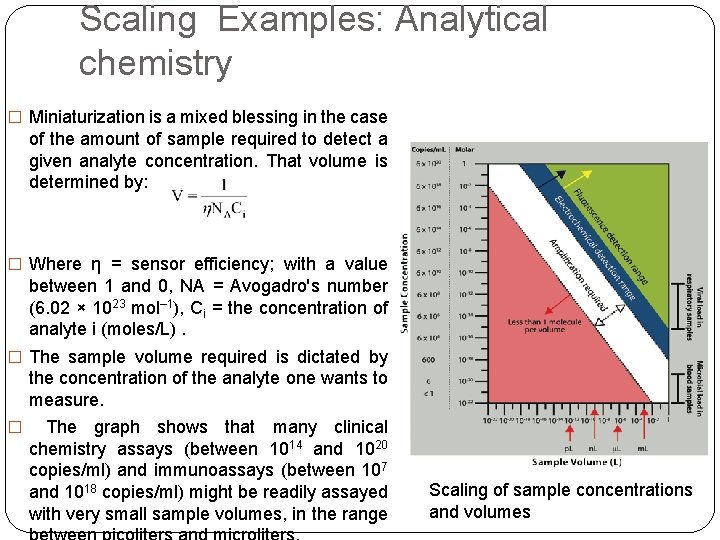
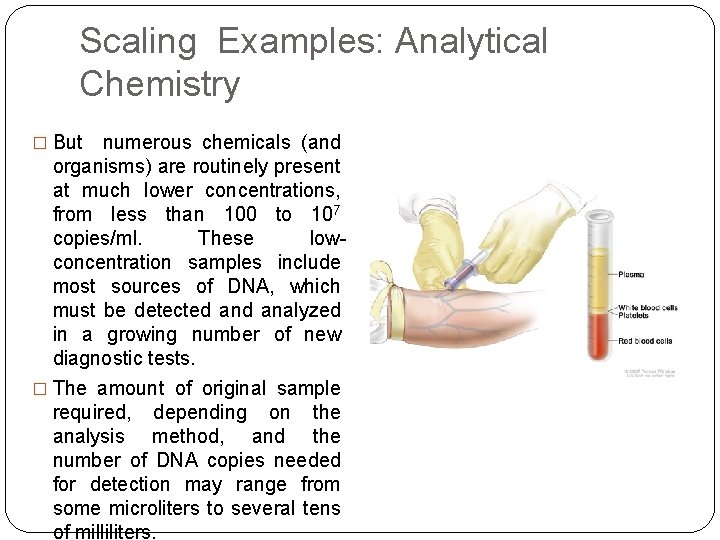
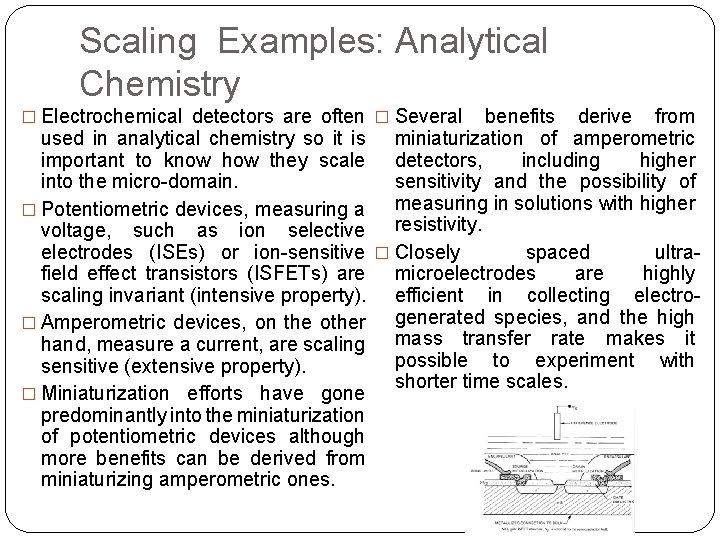
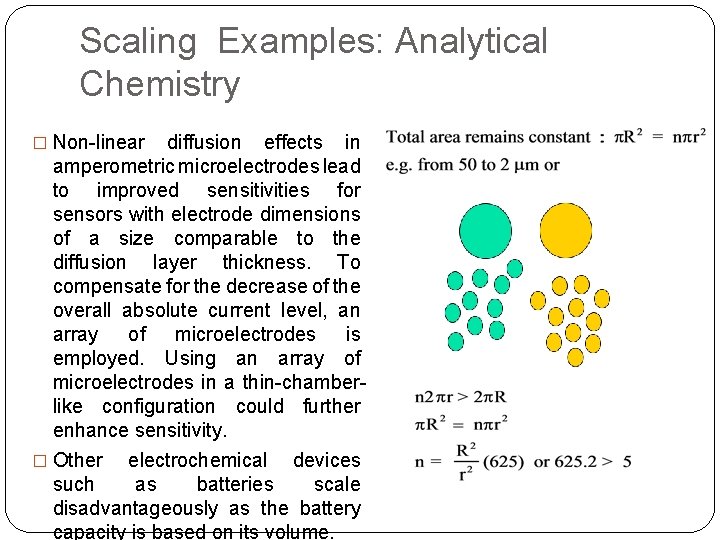
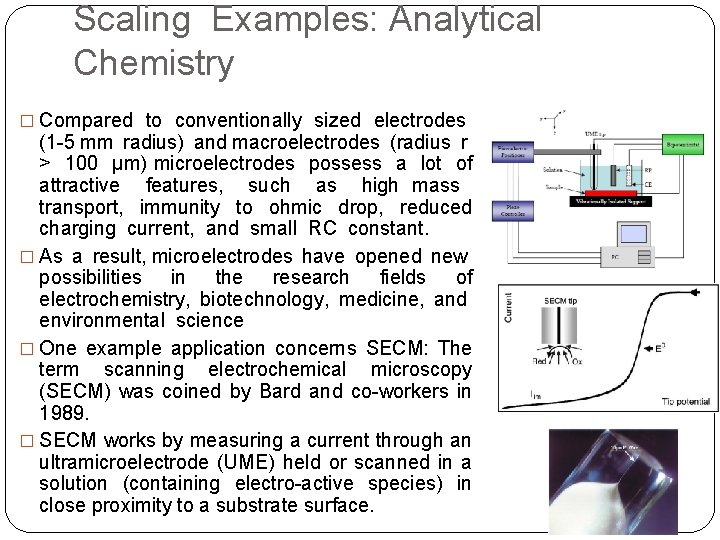
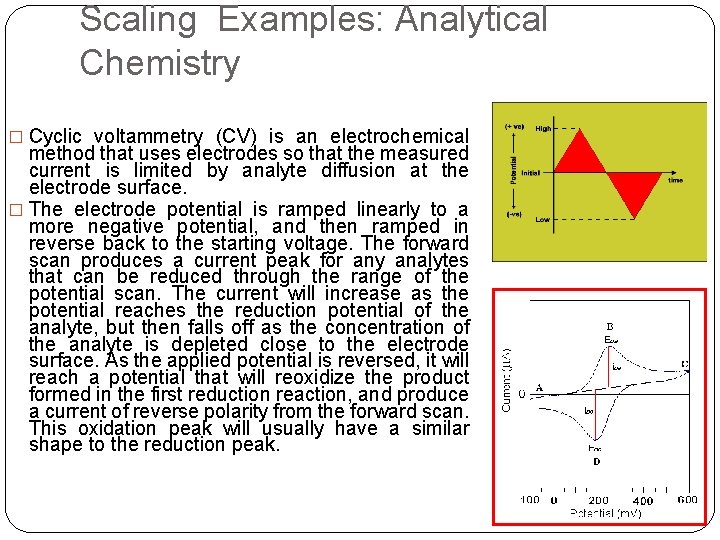
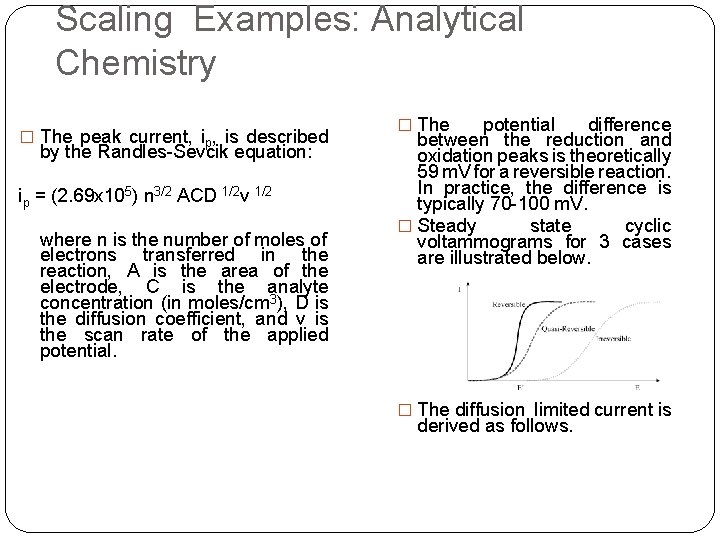
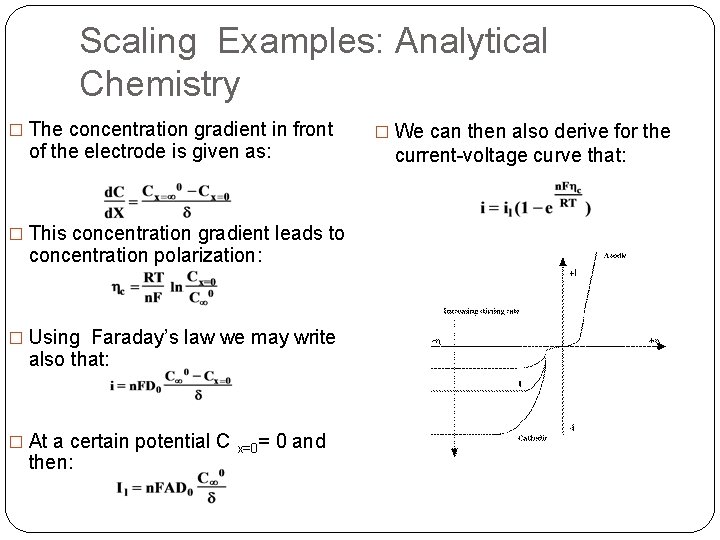
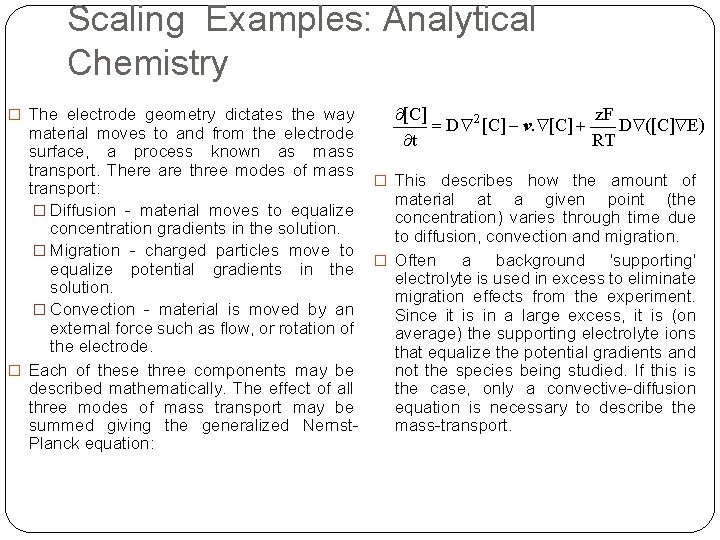
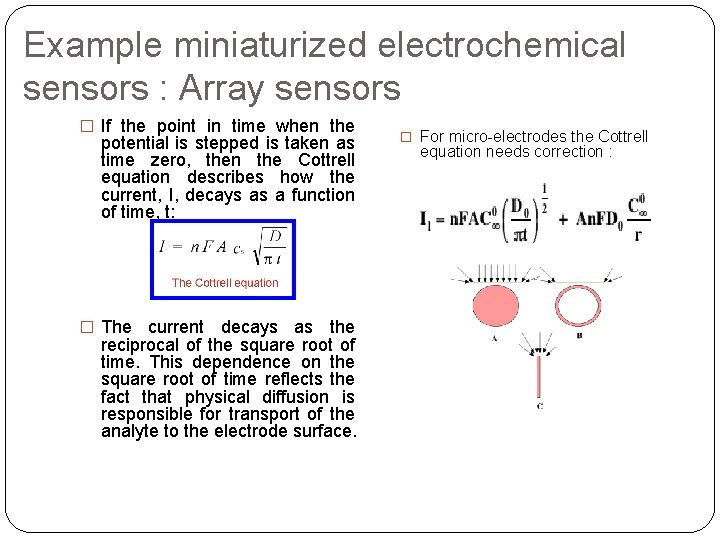
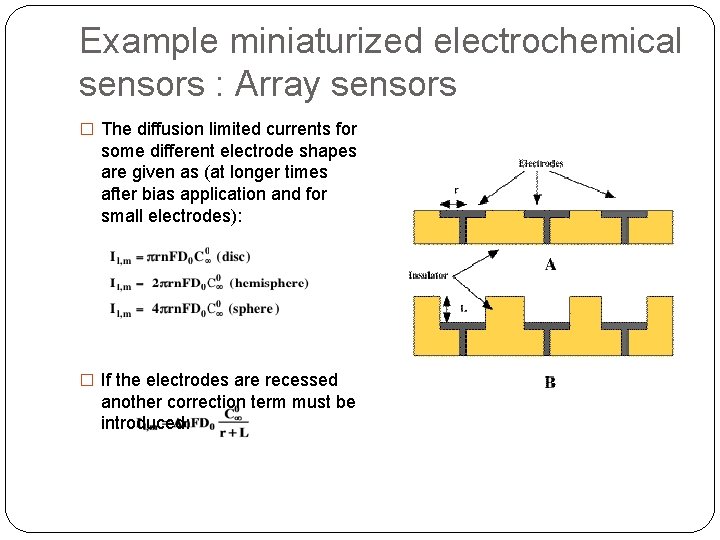
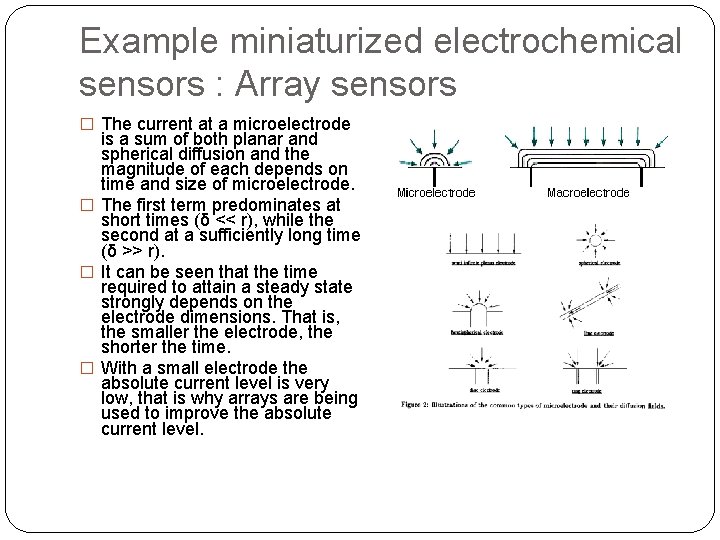
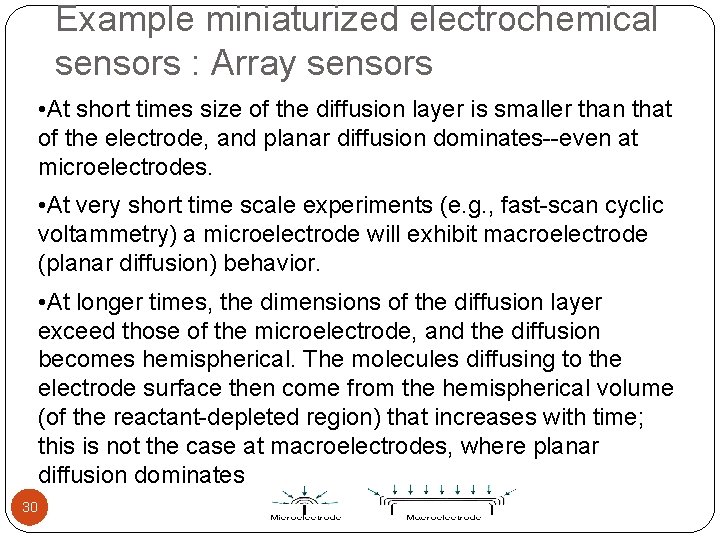
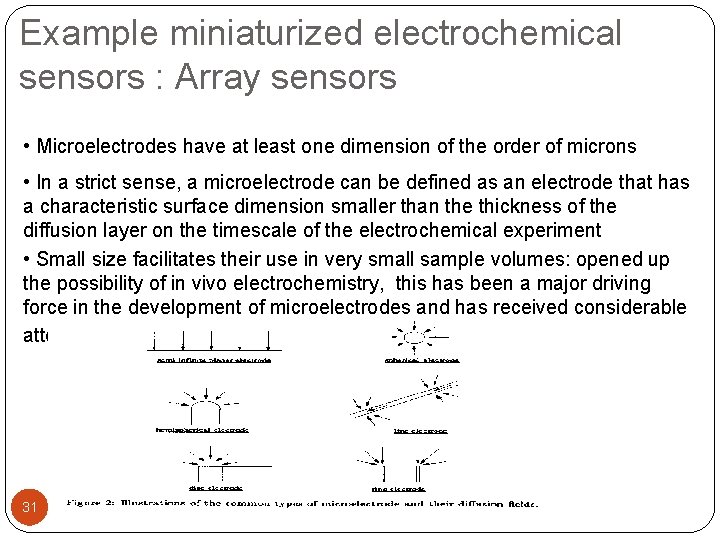
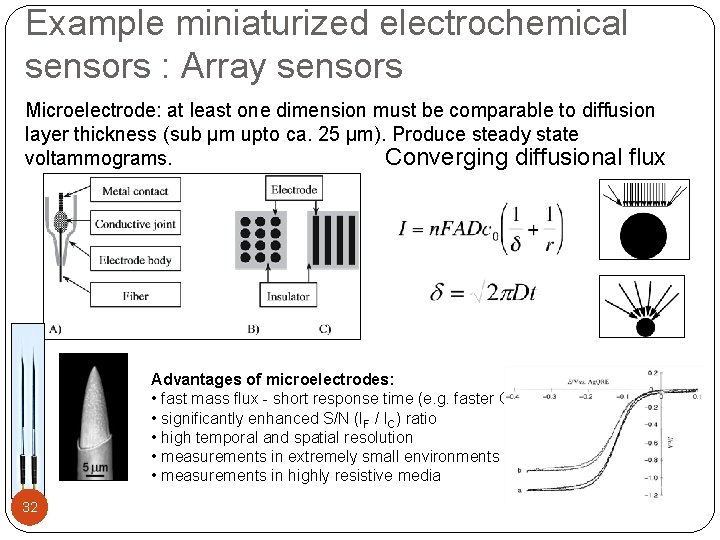
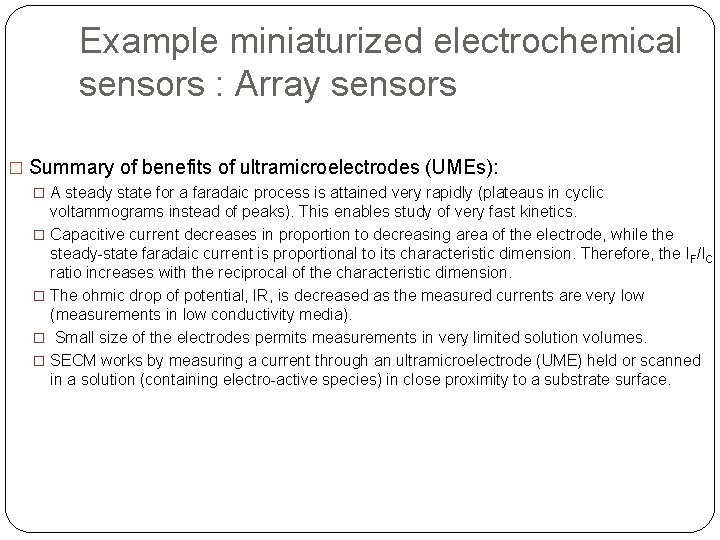
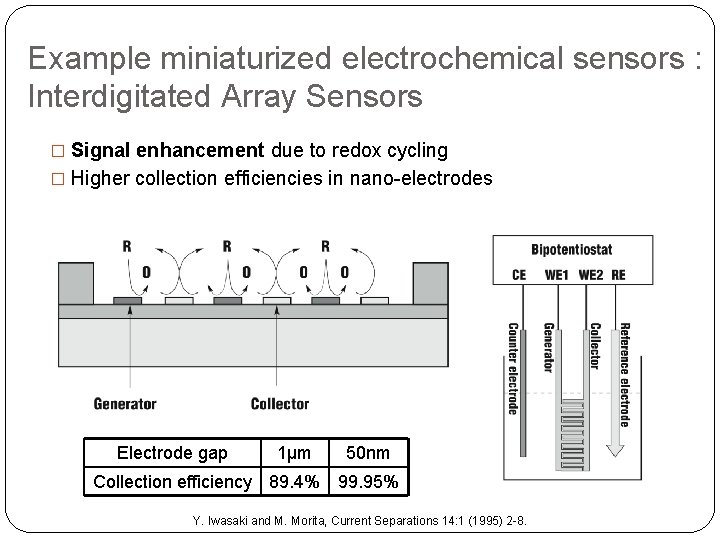
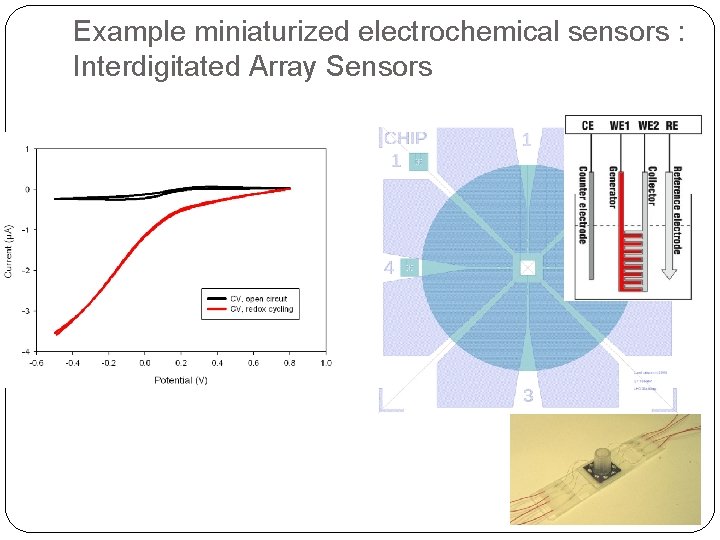
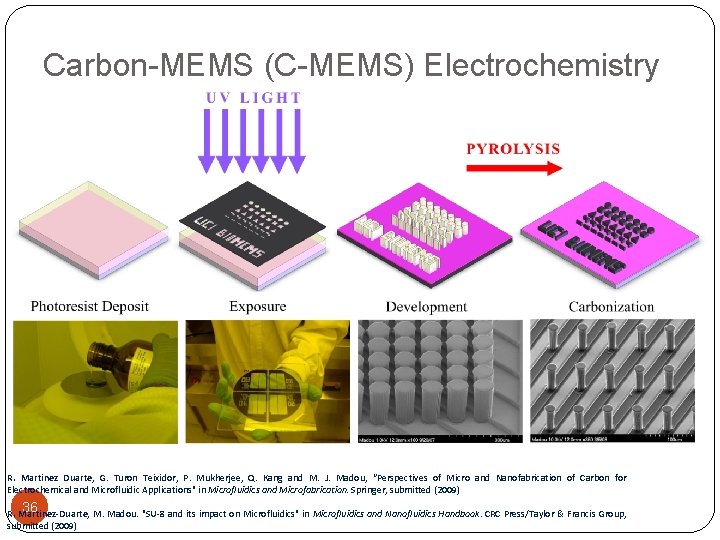
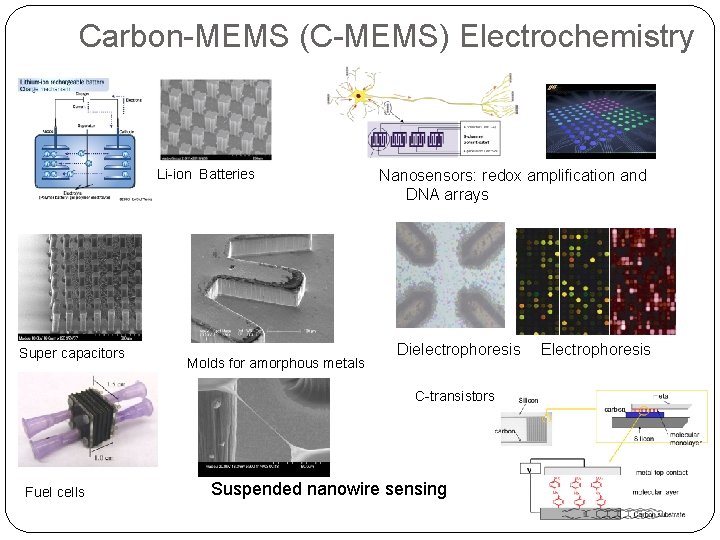
- Slides: 37
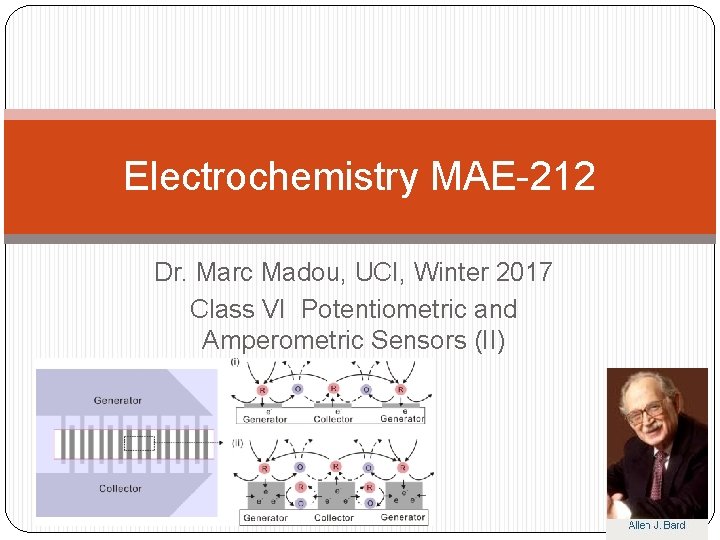
Electrochemistry MAE-212 Dr. Marc Madou, UCI, Winter 2017 Class VI Potentiometric and Amperometric Sensors (II)
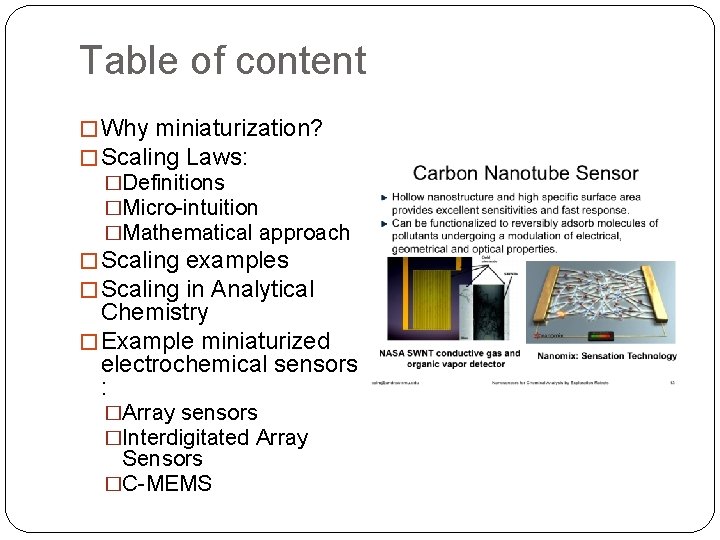
Table of content � Why miniaturization? � Scaling Laws: �Definitions �Micro-intuition �Mathematical approach � Scaling examples � Scaling in Analytical Chemistry � Example miniaturized electrochemical sensors : �Array sensors �Interdigitated Array Sensors �C-MEMS
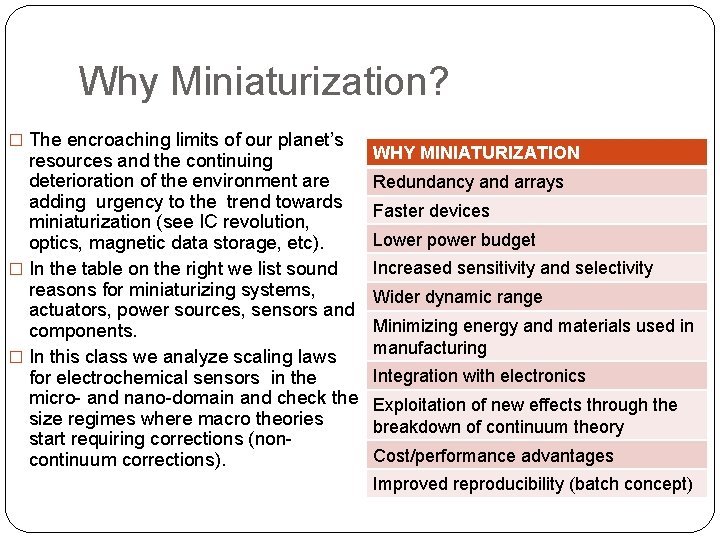
Why Miniaturization? � The encroaching limits of our planet’s resources and the continuing deterioration of the environment are adding urgency to the trend towards miniaturization (see IC revolution, optics, magnetic data storage, etc). � In the table on the right we list sound reasons for miniaturizing systems, actuators, power sources, sensors and components. � In this class we analyze scaling laws for electrochemical sensors in the micro- and nano-domain and check the size regimes where macro theories start requiring corrections (noncontinuum corrections). WHY MINIATURIZATION Redundancy and arrays Faster devices Lower power budget Increased sensitivity and selectivity Wider dynamic range Minimizing energy and materials used in manufacturing Integration with electronics Exploitation of new effects through the breakdown of continuum theory Cost/performance advantages Improved reproducibility (batch concept)
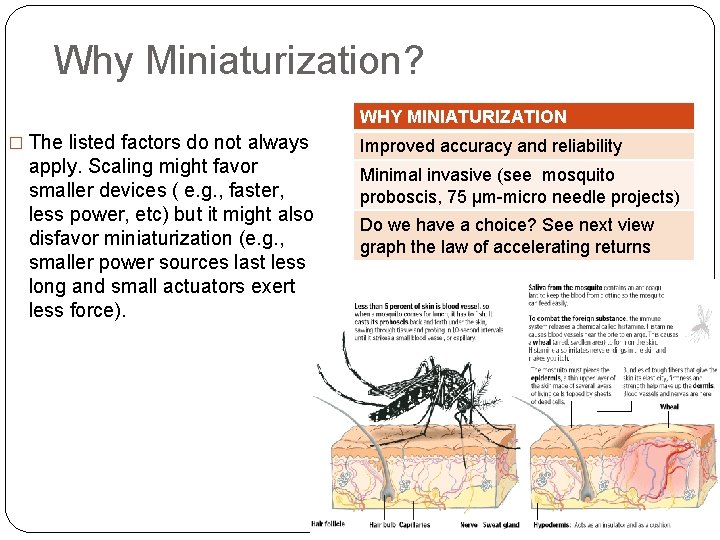
Why Miniaturization? WHY MINIATURIZATION � The listed factors do not always apply. Scaling might favor smaller devices ( e. g. , faster, less power, etc) but it might also disfavor miniaturization (e. g. , smaller power sources last less long and small actuators exert less force). Improved accuracy and reliability Minimal invasive (see mosquito proboscis, 75 µm-micro needle projects) Do we have a choice? See next view graph the law of accelerating returns
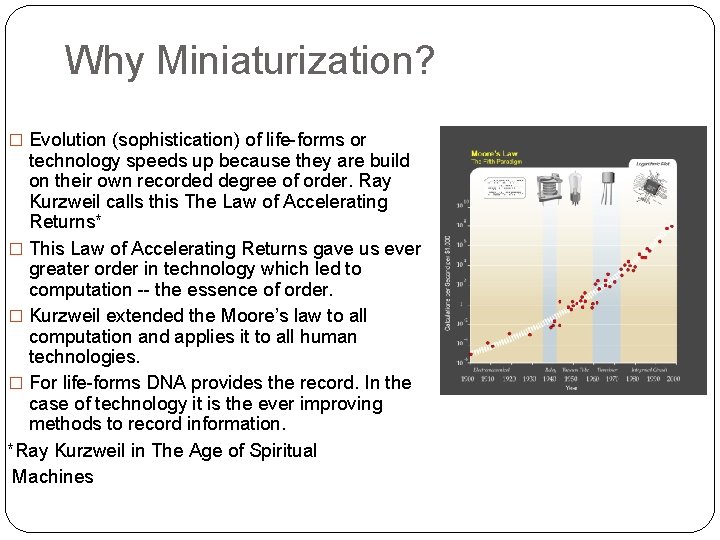
Why Miniaturization? � Evolution (sophistication) of life-forms or technology speeds up because they are build on their own recorded degree of order. Ray Kurzweil calls this The Law of Accelerating Returns* � This Law of Accelerating Returns gave us ever greater order in technology which led to computation -- the essence of order. � Kurzweil extended the Moore’s law to all computation and applies it to all human technologies. � For life-forms DNA provides the record. In the case of technology it is the ever improving methods to record information. *Ray Kurzweil in The Age of Spiritual Machines
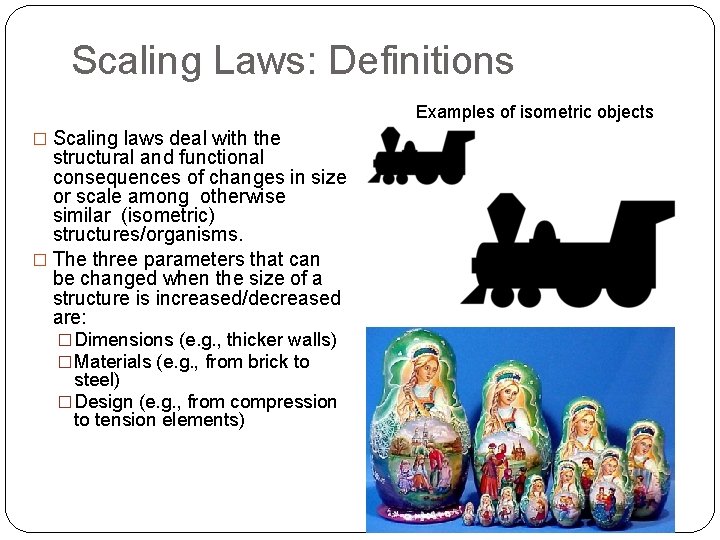
Scaling Laws: Definitions Examples of isometric objects � Scaling laws deal with the structural and functional consequences of changes in size or scale among otherwise similar (isometric) structures/organisms. � The three parameters that can be changed when the size of a structure is increased/decreased are: � Dimensions (e. g. , thicker walls) � Materials (e. g. , from brick to steel) � Design (e. g. , from compression to tension elements)
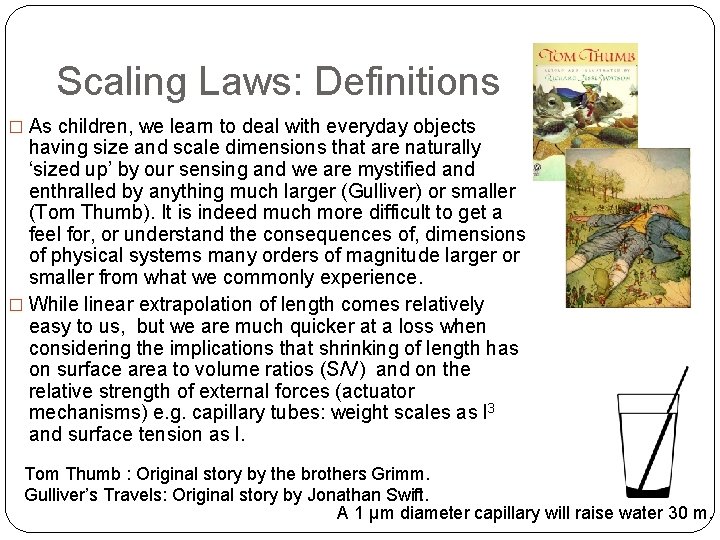
Scaling Laws: Definitions � As children, we learn to deal with everyday objects having size and scale dimensions that are naturally ‘sized up’ by our sensing and we are mystified and enthralled by anything much larger (Gulliver) or smaller (Tom Thumb). It is indeed much more difficult to get a feel for, or understand the consequences of, dimensions of physical systems many orders of magnitude larger or smaller from what we commonly experience. � While linear extrapolation of length comes relatively easy to us, but we are much quicker at a loss when considering the implications that shrinking of length has on surface area to volume ratios (S/V) and on the relative strength of external forces (actuator mechanisms) e. g. capillary tubes: weight scales as l 3 and surface tension as l. Tom Thumb : Original story by the brothers Grimm. Gulliver’s Travels: Original story by Jonathan Swift. A 1 µm diameter capillary will raise water 30 m.
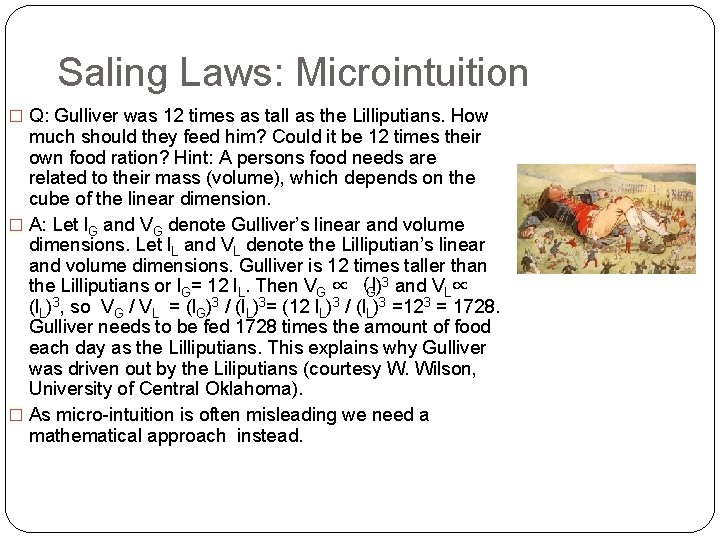
Saling Laws: Microintuition � Q: Gulliver was 12 times as tall as the Lilliputians. How much should they feed him? Could it be 12 times their own food ration? Hint: A persons food needs are related to their mass (volume), which depends on the cube of the linear dimension. � A: Let l. G and VG denote Gulliver’s linear and volume dimensions. Let l. L and VL denote the Lilliputian’s linear and volume dimensions. Gulliver is 12 times taller than 3 the Lilliputians or l. G= 12 l. L. Then VG ∝ (l G) and VL∝ (l. L)3, so VG / VL = (l. G)3 / (l. L)3= (12 l. L)3 / (l. L)3 =123 = 1728. Gulliver needs to be fed 1728 times the amount of food each day as the Lilliputians. This explains why Gulliver was driven out by the Liliputians (courtesy W. Wilson, University of Central Oklahoma). � As micro-intuition is often misleading we need a mathematical approach instead.
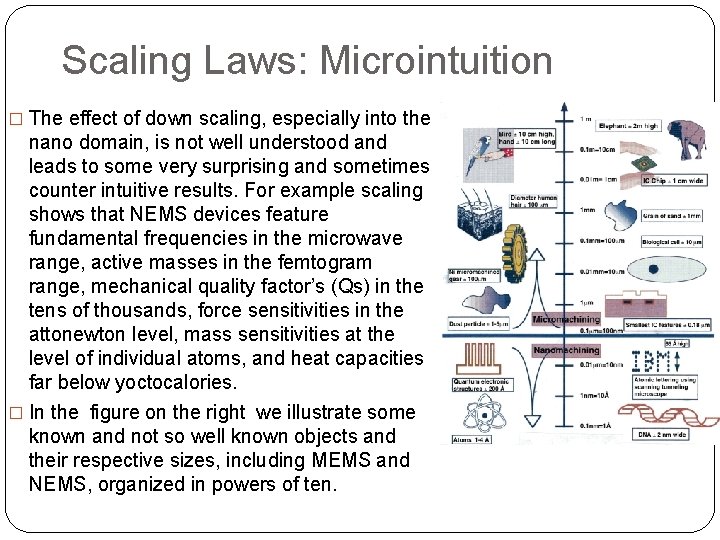
Scaling Laws: Microintuition � The effect of down scaling, especially into the nano domain, is not well understood and leads to some very surprising and sometimes counter intuitive results. For example scaling shows that NEMS devices feature fundamental frequencies in the microwave range, active masses in the femtogram range, mechanical quality factor’s (Qs) in the tens of thousands, force sensitivities in the attonewton level, mass sensitivities at the level of individual atoms, and heat capacities far below yoctocalories. � In the figure on the right we illustrate some known and not so well known objects and their respective sizes, including MEMS and NEMS, organized in powers of ten.
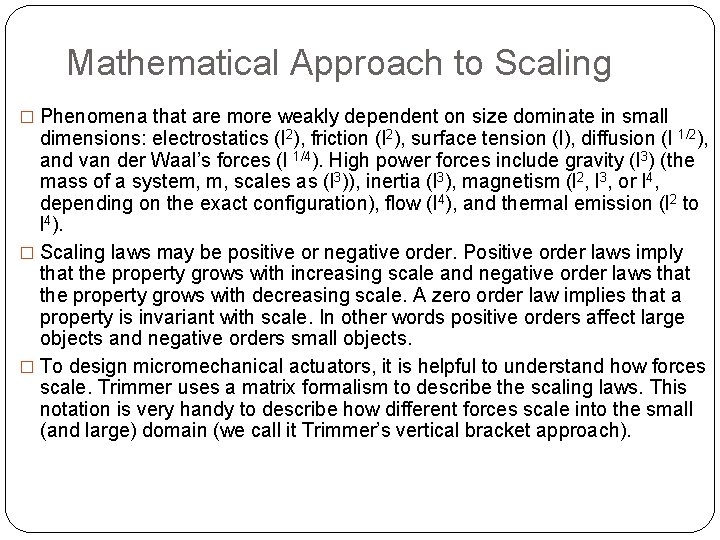
Mathematical Approach to Scaling � Phenomena that are more weakly dependent on size dominate in small dimensions: electrostatics (l 2), friction (l 2), surface tension (l), diffusion (l 1/2), and van der Waal’s forces (l 1/4). High power forces include gravity (l 3) (the mass of a system, m, scales as (l 3)), inertia (l 3), magnetism (l 2, l 3, or l 4, depending on the exact configuration), flow (l 4), and thermal emission (l 2 to l 4). � Scaling laws may be positive or negative order. Positive order laws imply that the property grows with increasing scale and negative order laws that the property grows with decreasing scale. A zero order law implies that a property is invariant with scale. In other words positive orders affect large objects and negative orders small objects. � To design micromechanical actuators, it is helpful to understand how forces scale. Trimmer uses a matrix formalism to describe the scaling laws. This notation is very handy to describe how different forces scale into the small (and large) domain (we call it Trimmer’s vertical bracket approach).
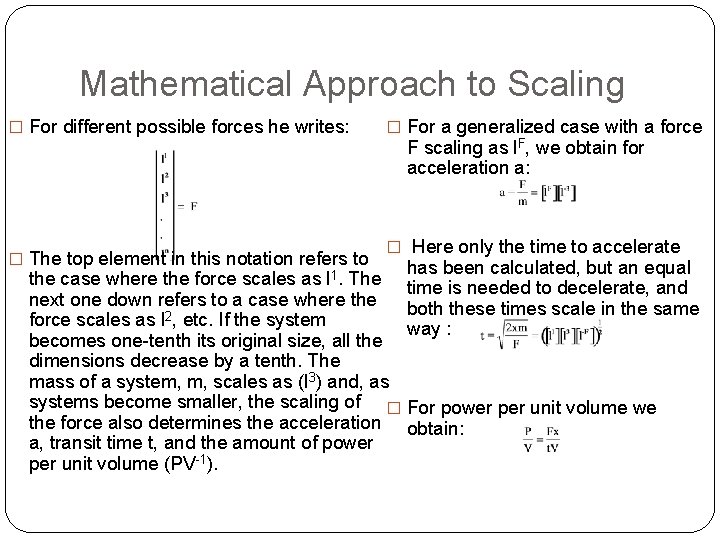
Mathematical Approach to Scaling � For different possible forces he writes: � The top element in this notation refers to � For a generalized case with a force F scaling as l. F, we obtain for acceleration a: � Here only the time to accelerate has been calculated, but an equal the case where the force scales as l 1. The time is needed to decelerate, and next one down refers to a case where the both these times scale in the same force scales as l 2, etc. If the system way : becomes one-tenth its original size, all the dimensions decrease by a tenth. The mass of a system, m, scales as (l 3) and, as systems become smaller, the scaling of � For power per unit volume we the force also determines the acceleration obtain: a, transit time t, and the amount of power per unit volume (PV-1).
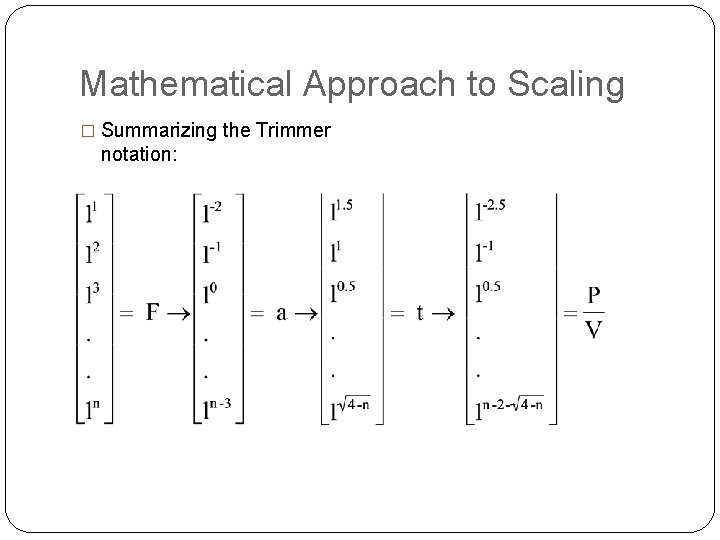
Mathematical Approach to Scaling � Summarizing the Trimmer notation:
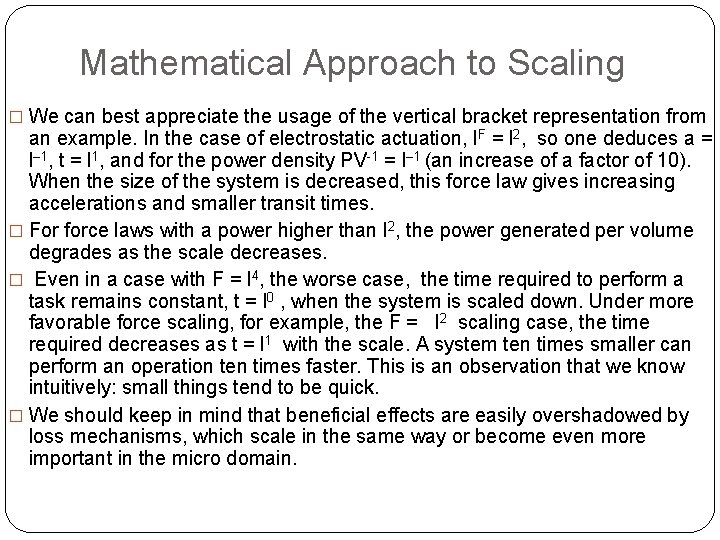
Mathematical Approach to Scaling � We can best appreciate the usage of the vertical bracket representation from an example. In the case of electrostatic actuation, l. F = l 2, so one deduces a = l– 1, t = l 1, and for the power density PV-1 = l– 1 (an increase of a factor of 10). When the size of the system is decreased, this force law gives increasing accelerations and smaller transit times. � For force laws with a power higher than l 2, the power generated per volume degrades as the scale decreases. � Even in a case with F = l 4, the worse case, the time required to perform a task remains constant, t = l 0 , when the system is scaled down. Under more favorable force scaling, for example, the F = l 2 scaling case, the time required decreases as t = l 1 with the scale. A system ten times smaller can perform an operation ten times faster. This is an observation that we know intuitively: small things tend to be quick. � We should keep in mind that beneficial effects are easily overshadowed by loss mechanisms, which scale in the same way or become even more important in the micro domain.
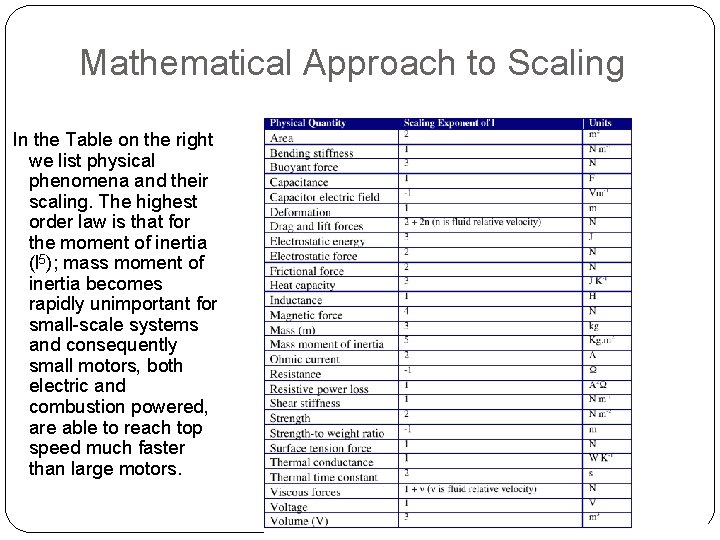
Mathematical Approach to Scaling In the Table on the right we list physical phenomena and their scaling. The highest order law is that for the moment of inertia (l 5); mass moment of inertia becomes rapidly unimportant for small-scale systems and consequently small motors, both electric and combustion powered, are able to reach top speed much faster than large motors.
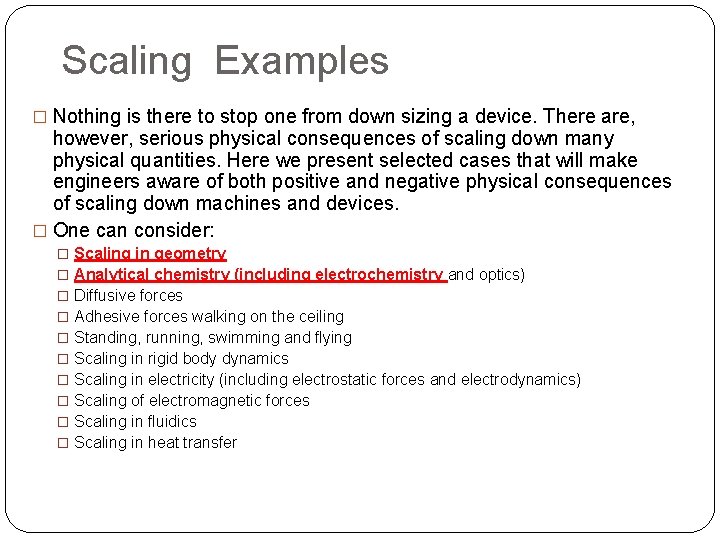
Scaling Examples � Nothing is there to stop one from down sizing a device. There are, however, serious physical consequences of scaling down many physical quantities. Here we present selected cases that will make engineers aware of both positive and negative physical consequences of scaling down machines and devices. � One can consider: � Scaling in geometry � Analytical chemistry (including electrochemistry and optics) � Diffusive forces � Adhesive forces walking on the ceiling � Standing, running, swimming and flying � Scaling in rigid body dynamics � Scaling in electricity (including electrostatic forces and electrodynamics) � Scaling of electromagnetic forces � Scaling in fluidics � Scaling in heat transfer
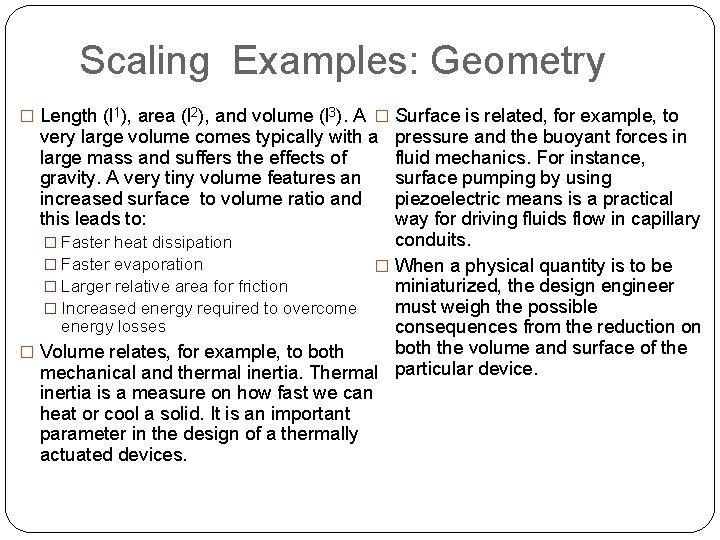
Scaling Examples: Geometry � Length (l 1), area (l 2), and volume (l 3). A � Surface is related, for example, to very large volume comes typically with a large mass and suffers the effects of gravity. A very tiny volume features an increased surface to volume ratio and this leads to: pressure and the buoyant forces in fluid mechanics. For instance, surface pumping by using piezoelectric means is a practical way for driving fluids flow in capillary conduits. � Faster heat dissipation � Faster evaporation � When a physical quantity is to be � Larger relative area for friction miniaturized, the design engineer must weigh the possible � Increased energy required to overcome energy losses consequences from the reduction on both the volume and surface of the � Volume relates, for example, to both mechanical and thermal inertia. Thermal particular device. inertia is a measure on how fast we can heat or cool a solid. It is an important parameter in the design of a thermally actuated devices.
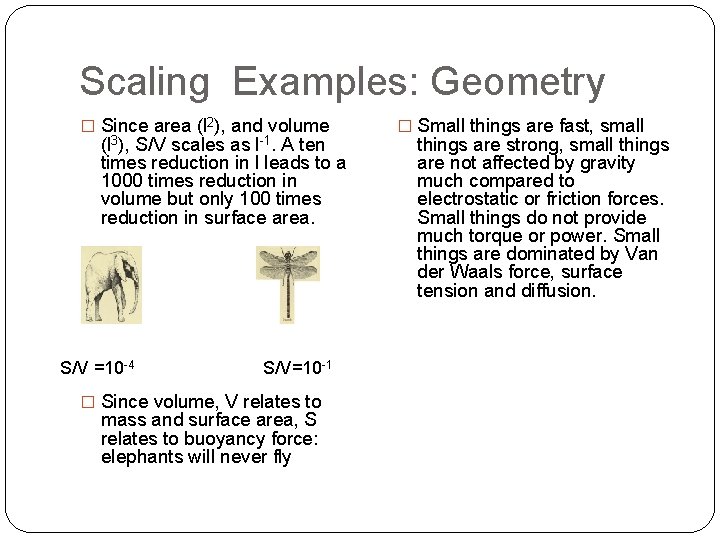
Scaling Examples: Geometry � Since area (l 2), and volume (l 3), S/V scales as A ten times reduction in l leads to a 1000 times reduction in volume but only 100 times reduction in surface area. S/V =10 -4 l-1. S/V=10 -1 � Since volume, V relates to mass and surface area, S relates to buoyancy force: elephants will never fly � Small things are fast, small things are strong, small things are not affected by gravity much compared to electrostatic or friction forces. Small things do not provide much torque or power. Small things are dominated by Van der Waals force, surface tension and diffusion.
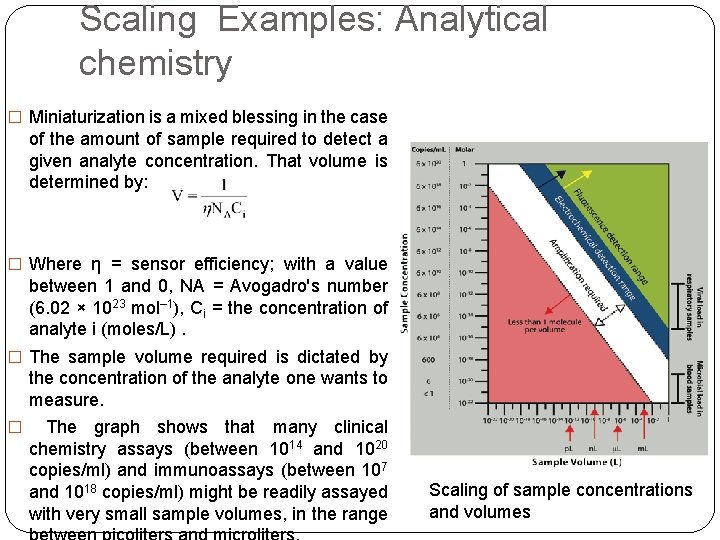
Scaling Examples: Analytical chemistry � Miniaturization is a mixed blessing in the case of the amount of sample required to detect a given analyte concentration. That volume is determined by: � Where η = sensor efficiency; with a value between 1 and 0, NA = Avogadro's number (6. 02 × 1023 mol– 1), Ci = the concentration of analyte i (moles/L). � The sample volume required is dictated by the concentration of the analyte one wants to measure. � The graph shows that many clinical chemistry assays (between 1014 and 1020 copies/ml) and immunoassays (between 107 and 1018 copies/ml) might be readily assayed with very small sample volumes, in the range Scaling of sample concentrations and volumes
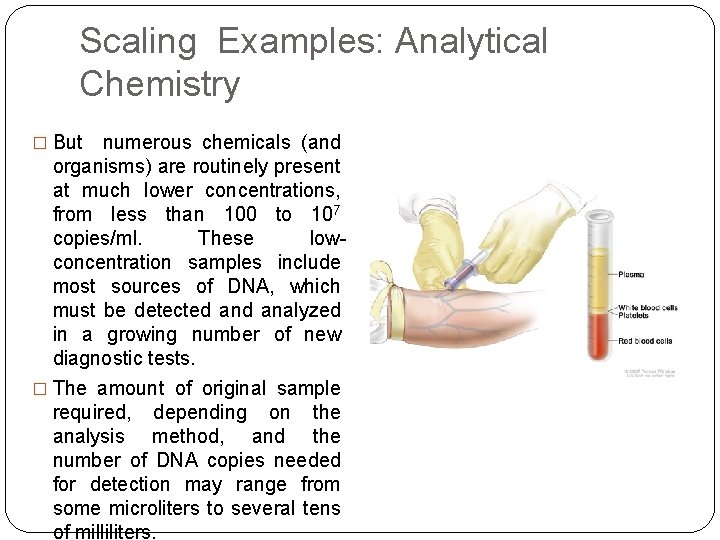
Scaling Examples: Analytical Chemistry � But numerous chemicals (and organisms) are routinely present at much lower concentrations, from less than 100 to 107 copies/ml. These lowconcentration samples include most sources of DNA, which must be detected analyzed in a growing number of new diagnostic tests. � The amount of original sample required, depending on the analysis method, and the number of DNA copies needed for detection may range from some microliters to several tens of milliliters.
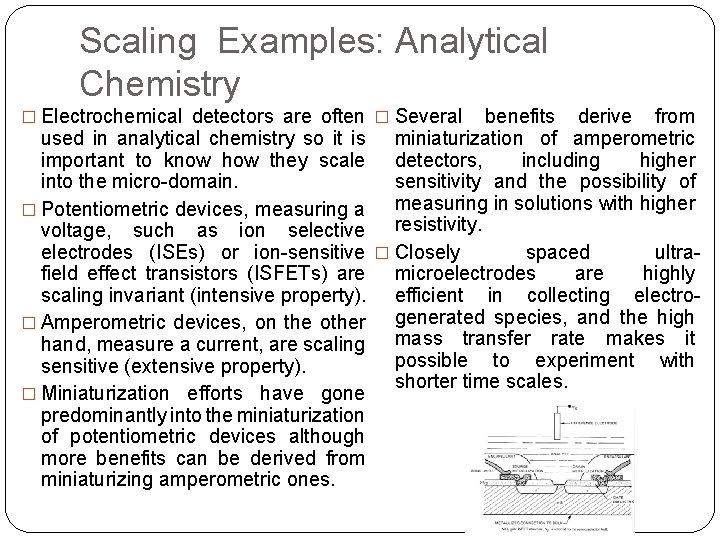
Scaling Examples: Analytical Chemistry � Electrochemical detectors are often � Several used in analytical chemistry so it is important to know how they scale into the micro-domain. � Potentiometric devices, measuring a voltage, such as ion selective electrodes (ISEs) or ion-sensitive field effect transistors (ISFETs) are scaling invariant (intensive property). � Amperometric devices, on the other hand, measure a current, are scaling sensitive (extensive property). � Miniaturization efforts have gone predominantly into the miniaturization of potentiometric devices although more benefits can be derived from miniaturizing amperometric ones. benefits derive from miniaturization of amperometric detectors, including higher sensitivity and the possibility of measuring in solutions with higher resistivity. � Closely spaced ultramicroelectrodes are highly efficient in collecting electrogenerated species, and the high mass transfer rate makes it possible to experiment with shorter time scales.
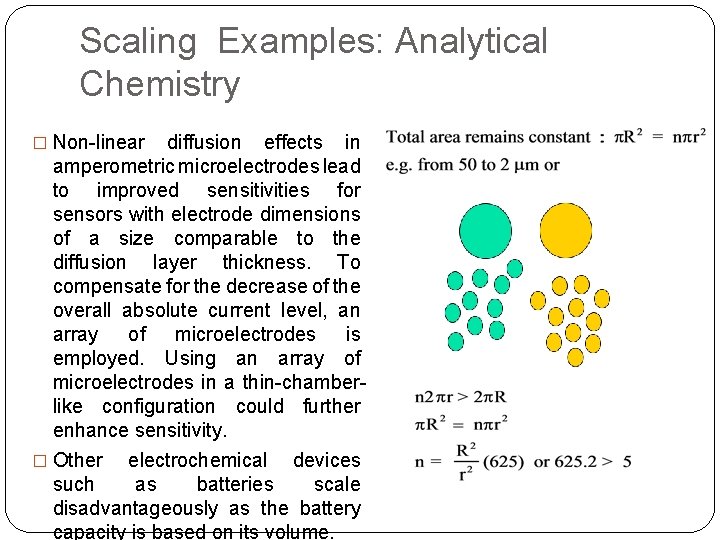
Scaling Examples: Analytical Chemistry � Non-linear diffusion effects in amperometric microelectrodes lead to improved sensitivities for sensors with electrode dimensions of a size comparable to the diffusion layer thickness. To compensate for the decrease of the overall absolute current level, an array of microelectrodes is employed. Using an array of microelectrodes in a thin-chamberlike configuration could further enhance sensitivity. � Other electrochemical devices such as batteries scale disadvantageously as the battery capacity is based on its volume.
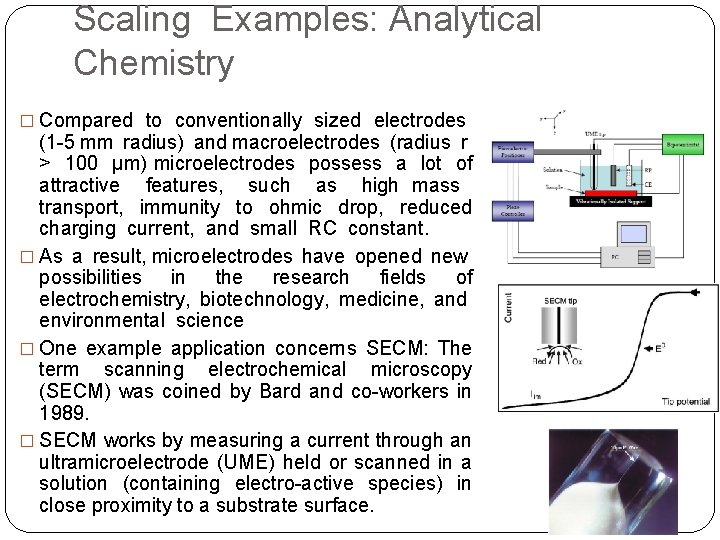
Scaling Examples: Analytical Chemistry � Compared to conventionally sized electrodes (1 -5 mm radius) and macroelectrodes (radius r > 100 μm) microelectrodes possess a lot of attractive features, such as high mass transport, immunity to ohmic drop, reduced charging current, and small RC constant. � As a result, microelectrodes have opened new possibilities in the research fields of electrochemistry, biotechnology, medicine, and environmental science � One example application concerns SECM: The term scanning electrochemical microscopy (SECM) was coined by Bard and co-workers in 1989. � SECM works by measuring a current through an ultramicroelectrode (UME) held or scanned in a solution (containing electro-active species) in close proximity to a substrate surface.
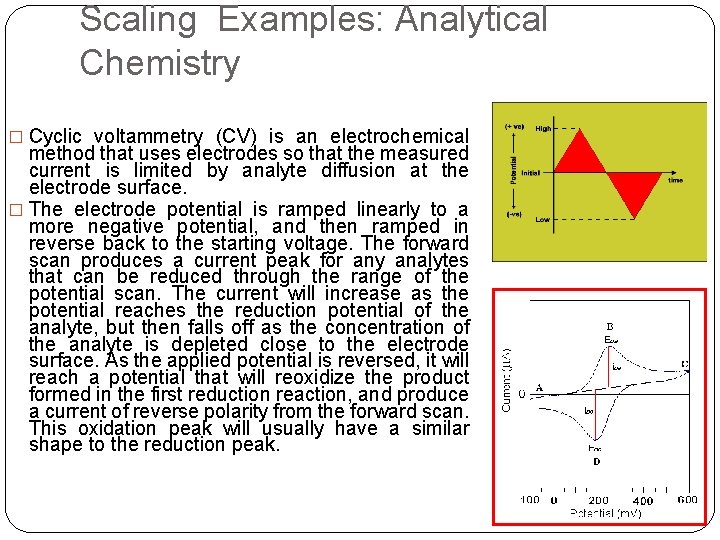
Scaling Examples: Analytical Chemistry � Cyclic voltammetry (CV) is an electrochemical method that uses electrodes so that the measured current is limited by analyte diffusion at the electrode surface. � The electrode potential is ramped linearly to a more negative potential, and then ramped in reverse back to the starting voltage. The forward scan produces a current peak for any analytes that can be reduced through the range of the potential scan. The current will increase as the potential reaches the reduction potential of the analyte, but then falls off as the concentration of the analyte is depleted close to the electrode surface. As the applied potential is reversed, it will reach a potential that will reoxidize the product formed in the first reduction reaction, and produce a current of reverse polarity from the forward scan. This oxidation peak will usually have a similar shape to the reduction peak.
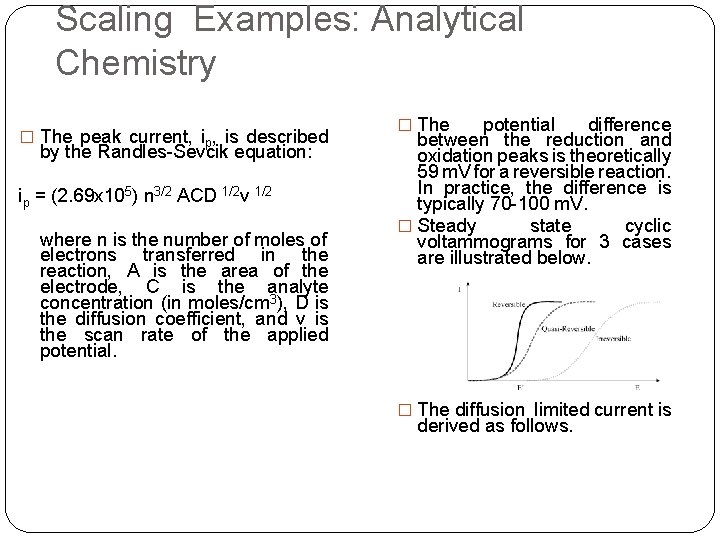
Scaling Examples: Analytical Chemistry � The peak current, ip, is described by the Randles-Sevcik equation: ip = (2. 69 x 105) n 3/2 ACD 1/2 v 1/2 where n is the number of moles of electrons transferred in the reaction, A is the area of the electrode, C is the analyte concentration (in moles/cm 3), D is the diffusion coefficient, and v is the scan rate of the applied potential. � The potential difference between the reduction and oxidation peaks is theoretically 59 m. V for a reversible reaction. In practice, the difference is typically 70 -100 m. V. � Steady state cyclic voltammograms for 3 cases are illustrated below. � The diffusion limited current is derived as follows.
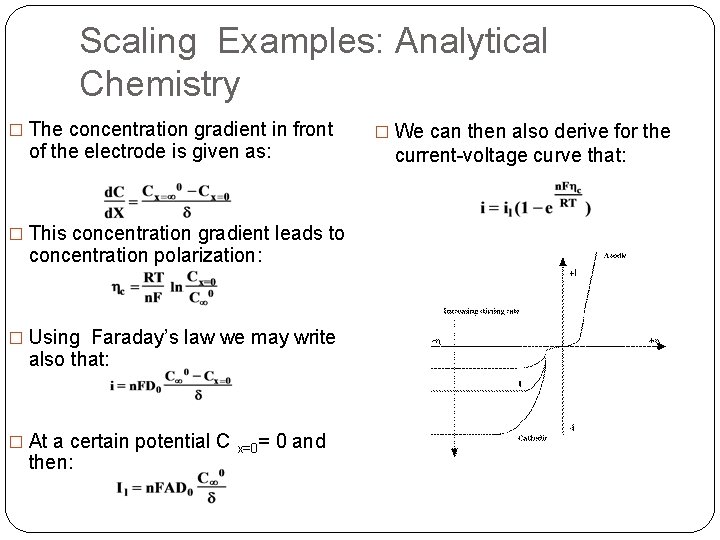
Scaling Examples: Analytical Chemistry � The concentration gradient in front of the electrode is given as: current-voltage curve that: � This concentration gradient leads to concentration polarization: � Using Faraday’s law we may write also that: � At a certain potential C then: x=0= � We can then also derive for the 0 and
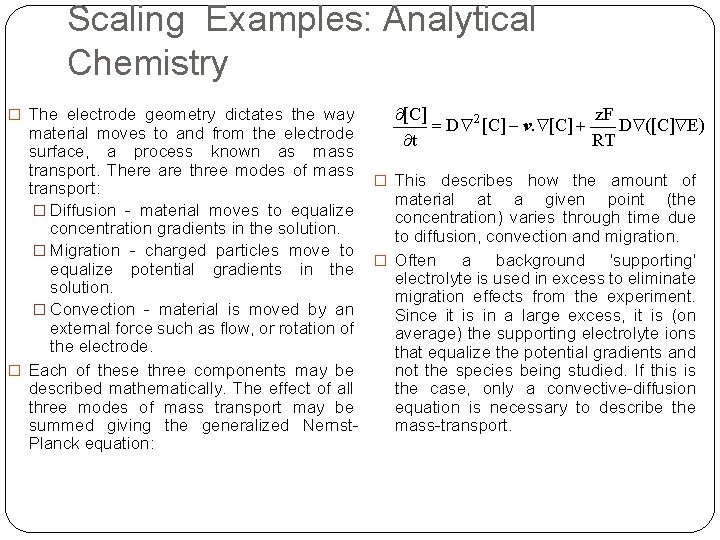
Scaling Examples: Analytical Chemistry � The electrode geometry dictates the way material moves to and from the electrode surface, a process known as mass transport. There are three modes of mass � This describes how the amount of transport: material at a given point (the � Diffusion - material moves to equalize concentration) varies through time due concentration gradients in the solution. to diffusion, convection and migration. � Migration - charged particles move to � Often a background 'supporting' equalize potential gradients in the electrolyte is used in excess to eliminate solution. migration effects from the experiment. � Convection - material is moved by an Since it is in a large excess, it is (on external force such as flow, or rotation of average) the supporting electrolyte ions the electrode. that equalize the potential gradients and not the species being studied. If this is � Each of these three components may be the case, only a convective-diffusion described mathematically. The effect of all equation is necessary to describe three modes of mass transport may be mass-transport. summed giving the generalized Nernst. Planck equation:
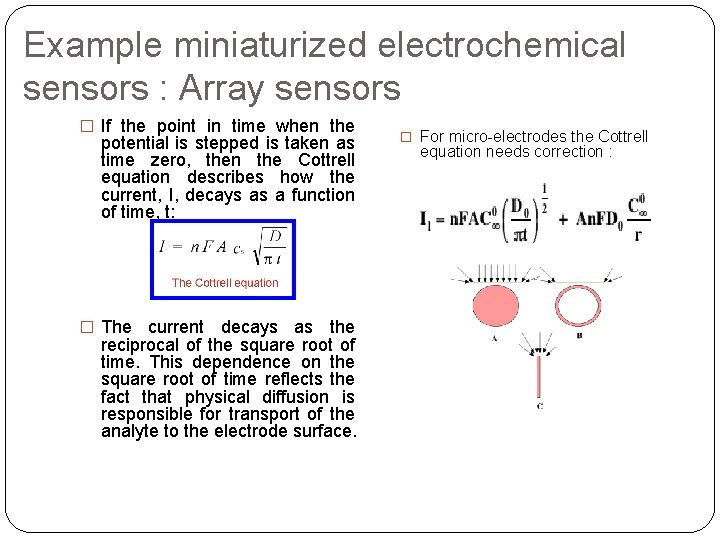
Example miniaturized electrochemical sensors : Array sensors � If the point in time when the potential is stepped is taken as time zero, then the Cottrell equation describes how the current, I, decays as a function of time, t: � The current decays as the reciprocal of the square root of time. This dependence on the square root of time reflects the fact that physical diffusion is responsible for transport of the analyte to the electrode surface. � For micro-electrodes the Cottrell equation needs correction :
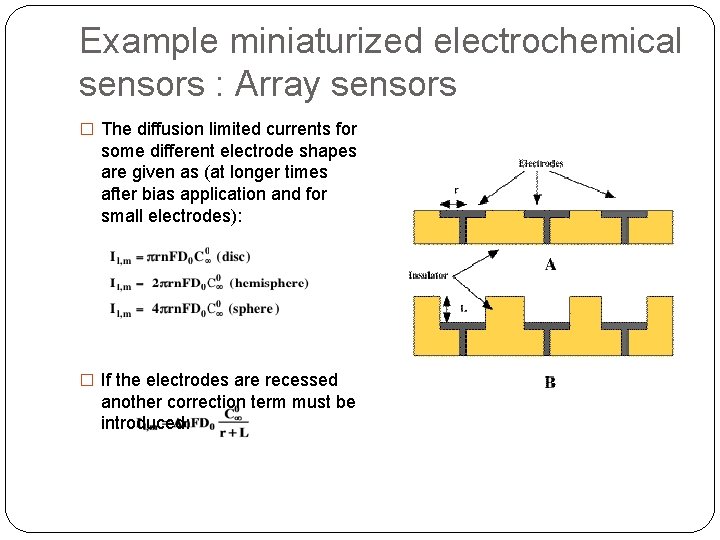
Example miniaturized electrochemical sensors : Array sensors � The diffusion limited currents for some different electrode shapes are given as (at longer times after bias application and for small electrodes): � If the electrodes are recessed another correction term must be introduced:
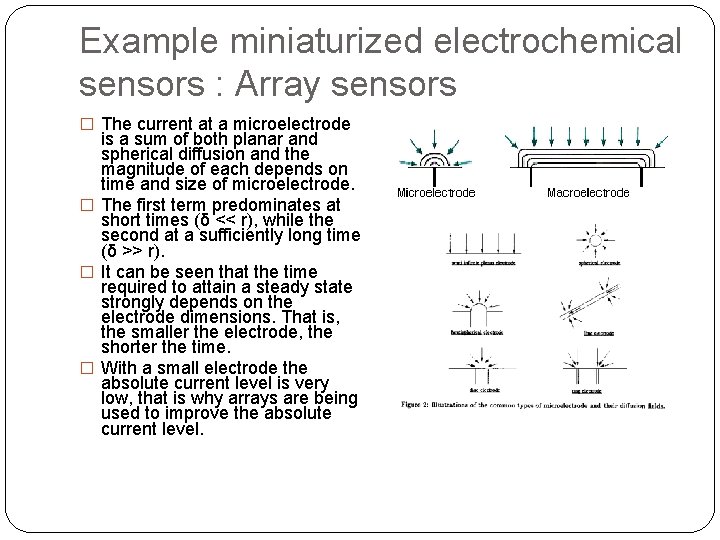
Example miniaturized electrochemical sensors : Array sensors � The current at a microelectrode is a sum of both planar and spherical diffusion and the magnitude of each depends on time and size of microelectrode. � The first term predominates at short times (δ << r), while the second at a sufficiently long time (δ >> r). � It can be seen that the time required to attain a steady state strongly depends on the electrode dimensions. That is, the smaller the electrode, the shorter the time. � With a small electrode the absolute current level is very low, that is why arrays are being used to improve the absolute current level.
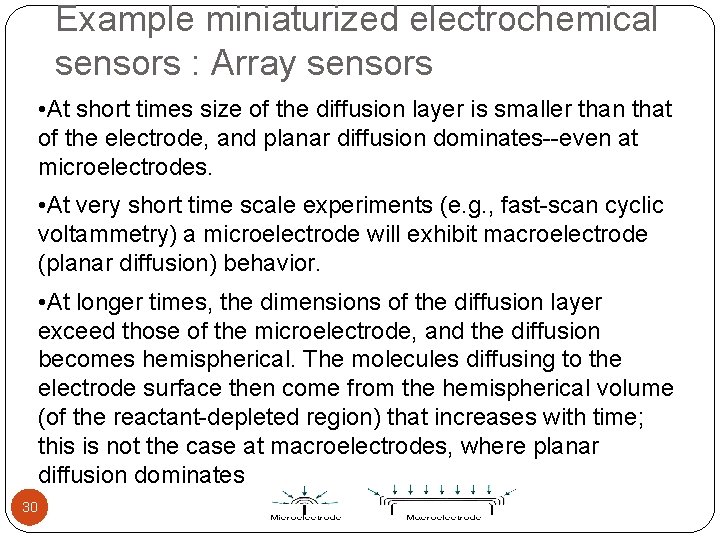
Example miniaturized electrochemical sensors : Array sensors • At short times size of the diffusion layer is smaller than that of the electrode, and planar diffusion dominates--even at microelectrodes. • At very short time scale experiments (e. g. , fast-scan cyclic voltammetry) a microelectrode will exhibit macroelectrode (planar diffusion) behavior. • At longer times, the dimensions of the diffusion layer exceed those of the microelectrode, and the diffusion becomes hemispherical. The molecules diffusing to the electrode surface then come from the hemispherical volume (of the reactant-depleted region) that increases with time; this is not the case at macroelectrodes, where planar diffusion dominates 30
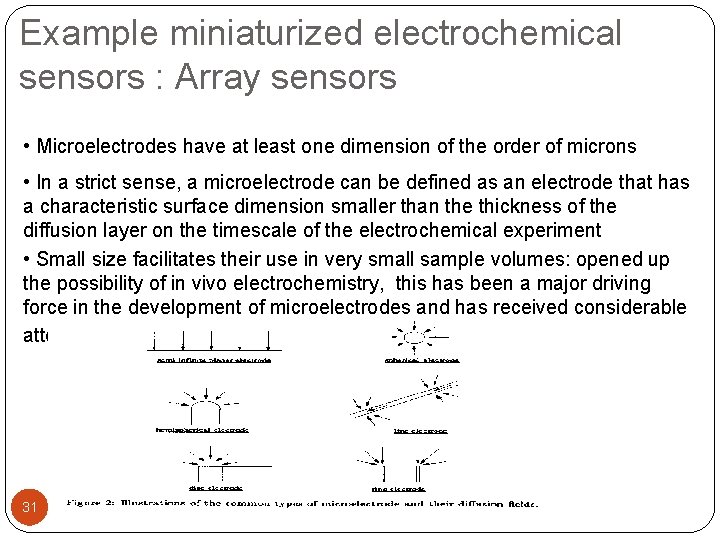
Example miniaturized electrochemical sensors : Array sensors • Microelectrodes have at least one dimension of the order of microns • In a strict sense, a microelectrode can be defined as an electrode that has a characteristic surface dimension smaller than the thickness of the diffusion layer on the timescale of the electrochemical experiment • Small size facilitates their use in very small sample volumes: opened up the possibility of in vivo electrochemistry, this has been a major driving force in the development of microelectrodes and has received considerable attention. 31
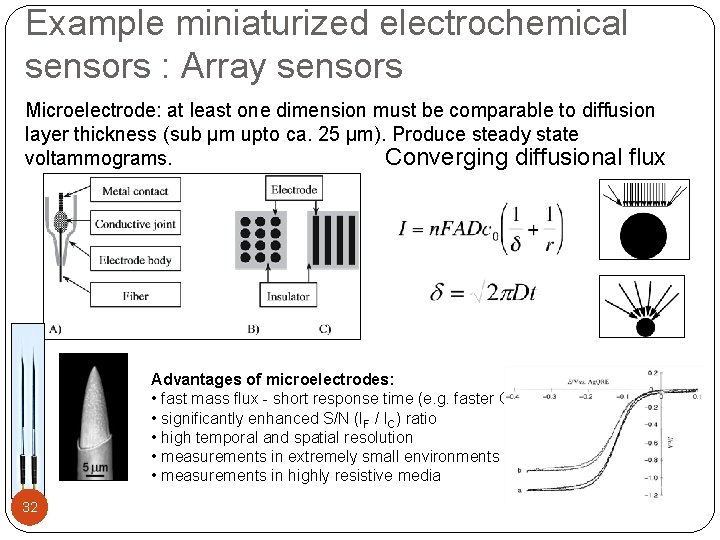
Example miniaturized electrochemical sensors : Array sensors Microelectrode: at least one dimension must be comparable to diffusion layer thickness (sub μm upto ca. 25 μm). Produce steady state voltammograms. Converging diffusional flux Advantages of microelectrodes: • fast mass flux - short response time (e. g. faster CV) • significantly enhanced S/N (IF / IC) ratio • high temporal and spatial resolution • measurements in extremely small environments • measurements in highly resistive media 32
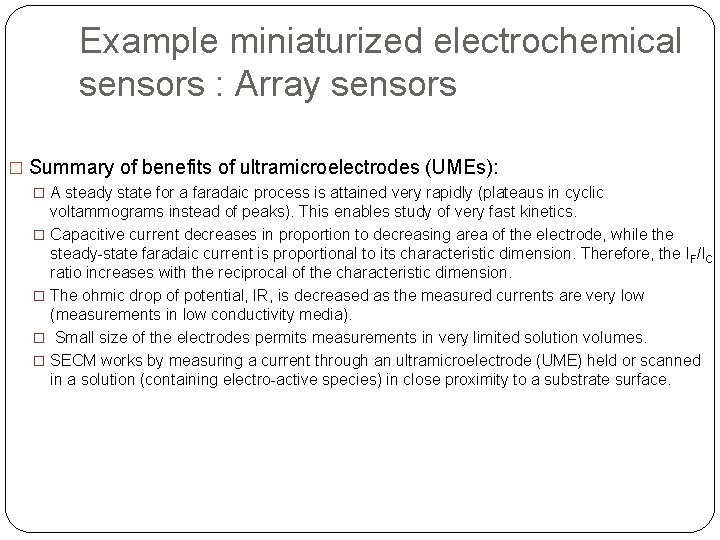
Example miniaturized electrochemical sensors : Array sensors � Summary of benefits of ultramicroelectrodes (UMEs): � A steady state for a faradaic process is attained very rapidly (plateaus in cyclic voltammograms instead of peaks). This enables study of very fast kinetics. � Capacitive current decreases in proportion to decreasing area of the electrode, while the steady-state faradaic current is proportional to its characteristic dimension. Therefore, the IF/IC ratio increases with the reciprocal of the characteristic dimension. � The ohmic drop of potential, IR, is decreased as the measured currents are very low (measurements in low conductivity media). � Small size of the electrodes permits measurements in very limited solution volumes. � SECM works by measuring a current through an ultramicroelectrode (UME) held or scanned in a solution (containing electro-active species) in close proximity to a substrate surface.
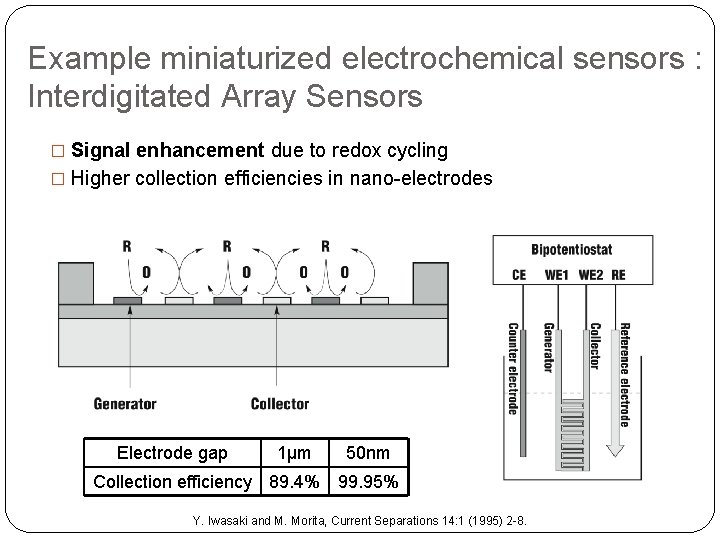
Example miniaturized electrochemical sensors : Interdigitated Array Sensors � Signal enhancement due to redox cycling � Higher collection efficiencies in nano-electrodes Electrode gap 1µm 50 nm Collection efficiency 89. 4% 99. 95% Y. Iwasaki and M. Morita, Current Separations 14: 1 (1995) 2 -8.
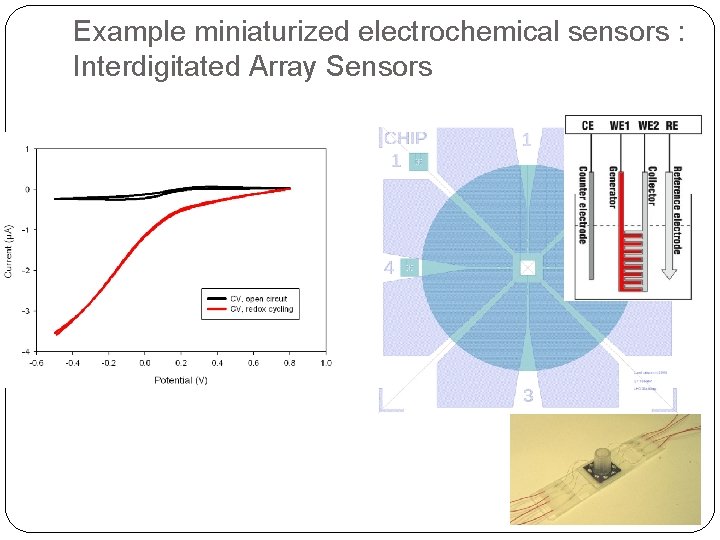
Example miniaturized electrochemical sensors : Interdigitated Array Sensors
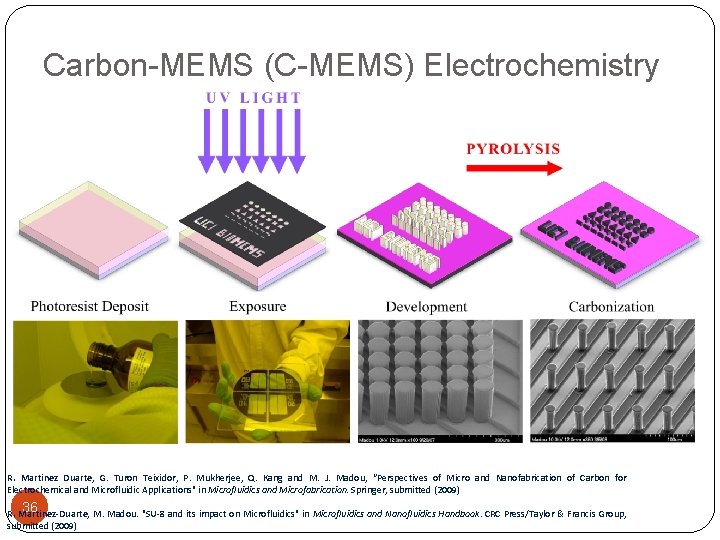
Carbon-MEMS (C-MEMS) Electrochemistry R. Martinez Duarte, G. Turon Teixidor, P. Mukherjee, Q. Kang and M. J. Madou, “Perspectives of Micro and Nanofabrication of Carbon for Electrochemical and Microfluidic Applications" in Microfluidics and Microfabrication. Springer, submitted (2009) 36 R. Martinez-Duarte, M. Madou. "SU-8 and its impact on Microfluidics" in Microfluidics and Nanofluidics Handbook. CRC Press/Taylor & Francis Group, submitted (2009)
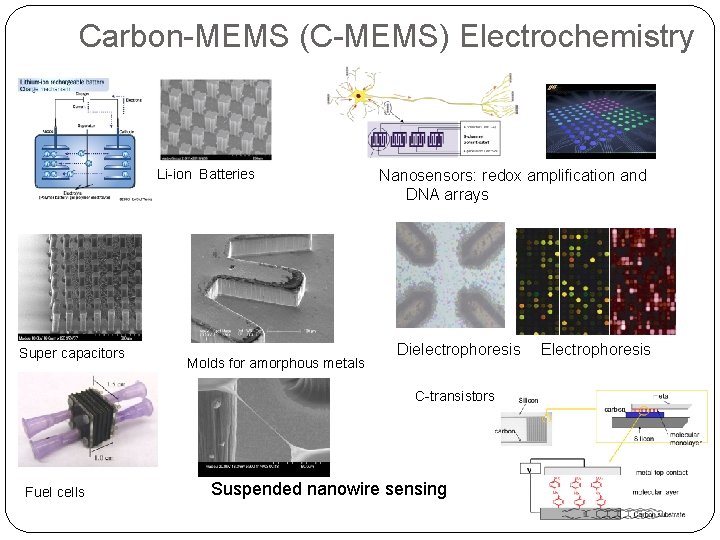
Carbon-MEMS (C-MEMS) Electrochemistry Li-ion Batteries Super capacitors Molds for amorphous metals Nanosensors: redox amplification and DNA arrays Dielectrophoresis C-transistors Fuel cells Suspended nanowire sensing Electrophoresis
Belfius madou
Winter kommt winter kommt flocken fallen nieder
Es war eine mutter
Winter kommt winter kommt flocken fallen nieder
Electrochemistry balancing equations
Electrochemistry ap chemistry
Polarization in electrochemistry
What is electrochemistry
Electroanalytical methods
Concentration cell
Chapter 20 electrochemistry
Electrolytic cell khan academy
Electrochemistry
What is electrochemistry in chemistry
Junction potential
Half redox reaction
Analytical electrochemistry
Electrochemistry balancing equations
Mass transport electrochemistry
Electrochemistry lesson plan
Electrochemistry eds
Balancing complex redox reactions
Electrochemistry
Ir drop
Diagonal rule electrochemistry
Line notation electrochemistry
Ap chemistry chapter 18 electrochemistry test
Cell chapter 21
Red cat electrochemistry
Electrochemistry stoichiometry
Electroanalytical techniques
Faraday's constant
Fundamentals of electrochemistry
Chapter 20 review electrochemistry
Voltaic vs electrolytic cell
Coupon technology
Diagonal rule electrochemistry
Concentration cell