44 Neurons Glia and Nervous Systems 44 Neurons
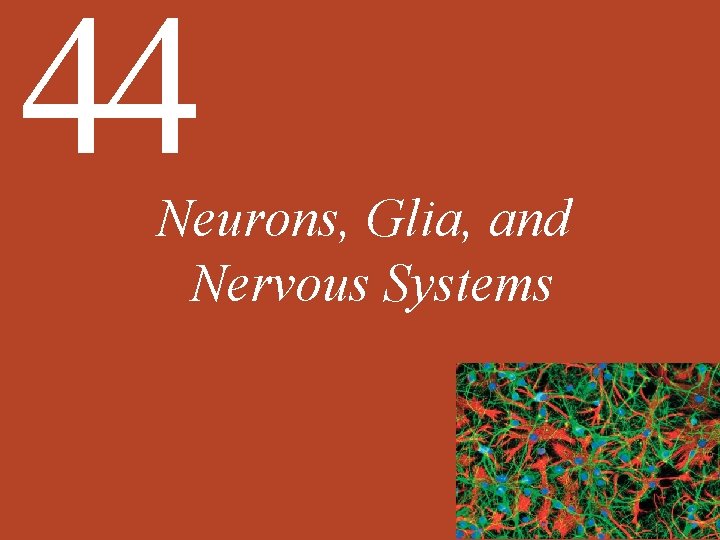
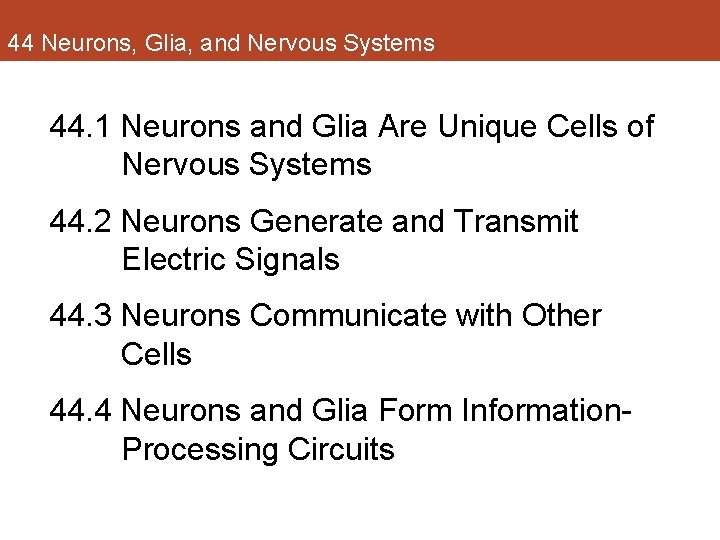
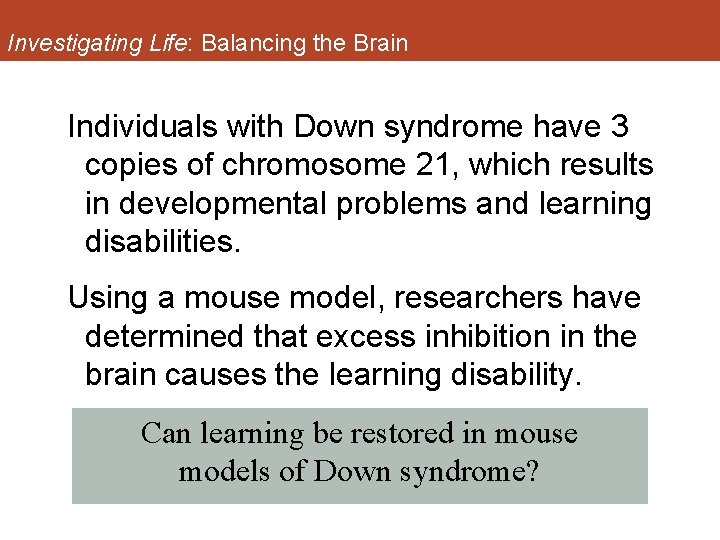
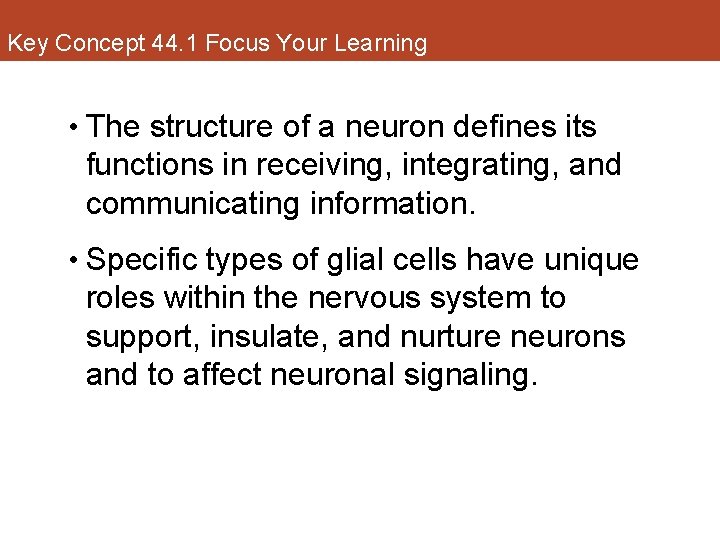
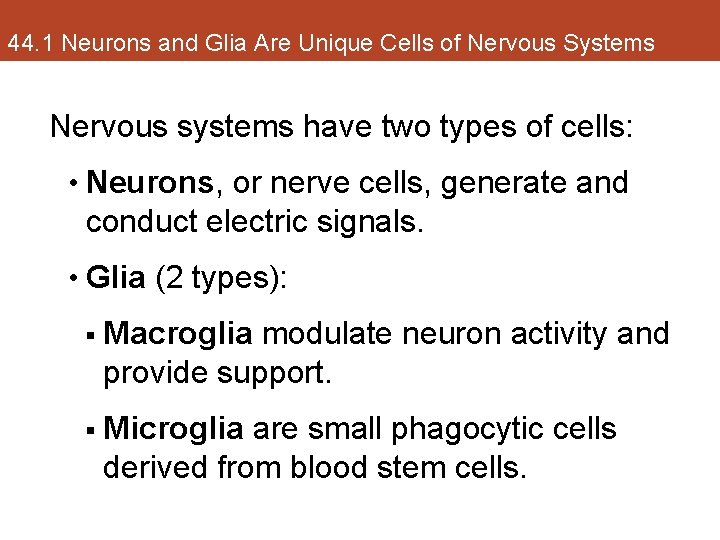
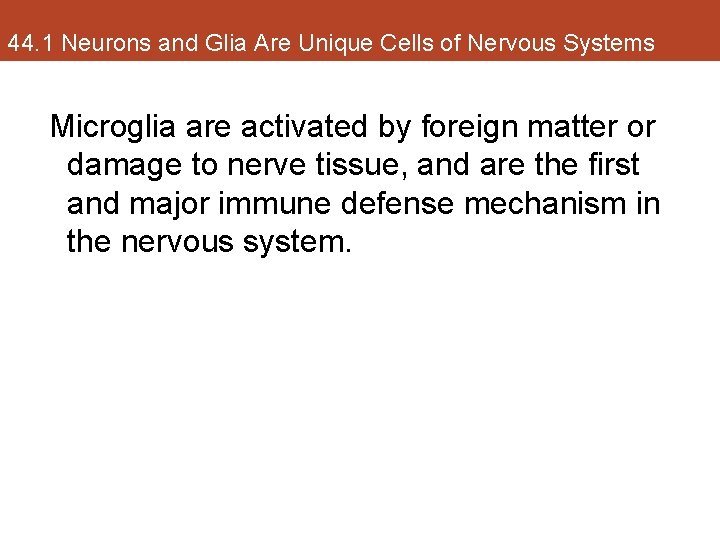
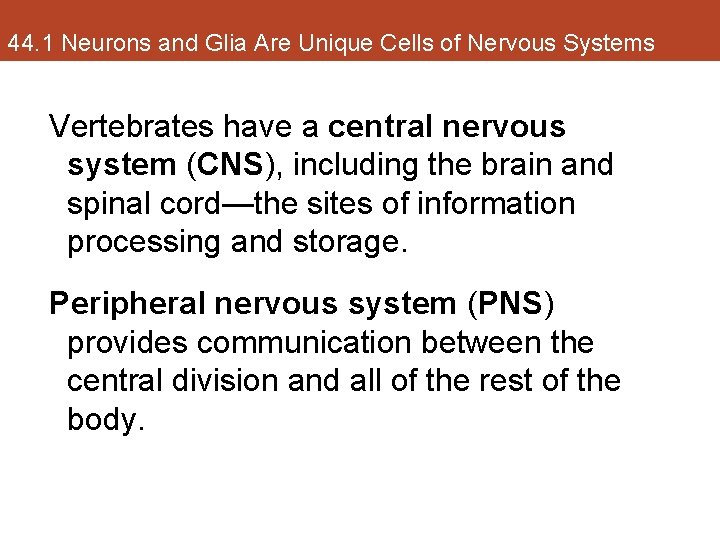
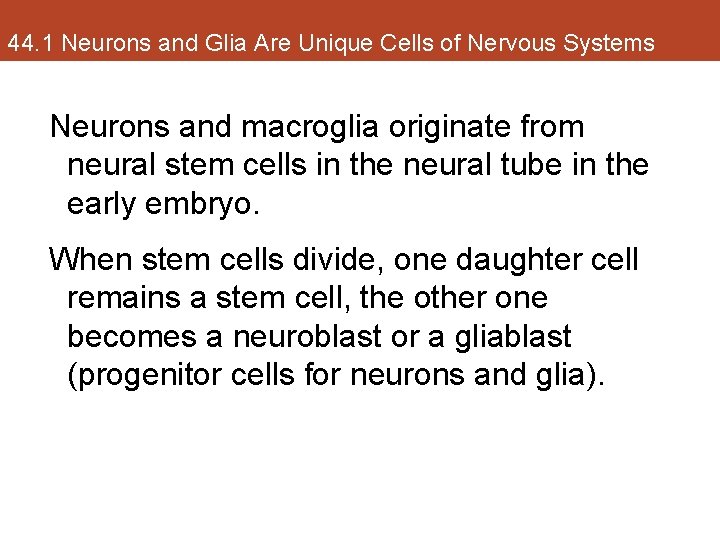
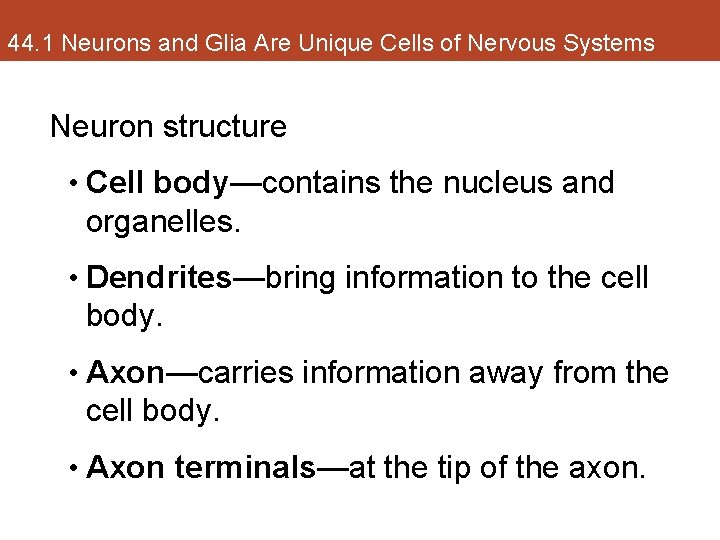
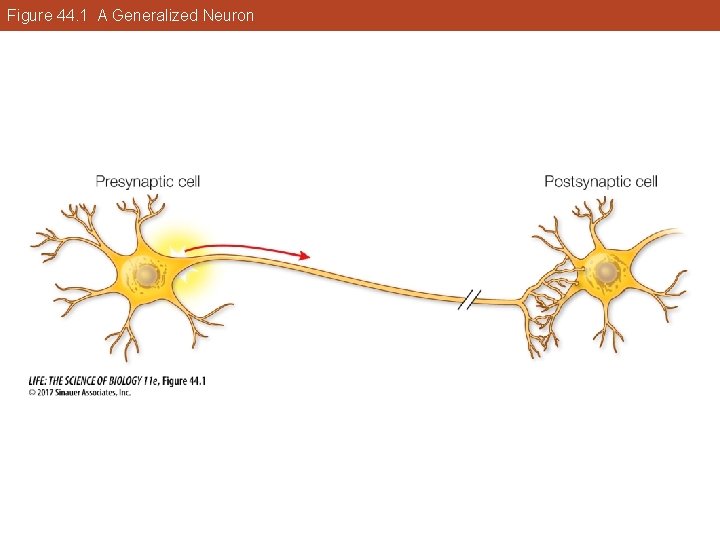
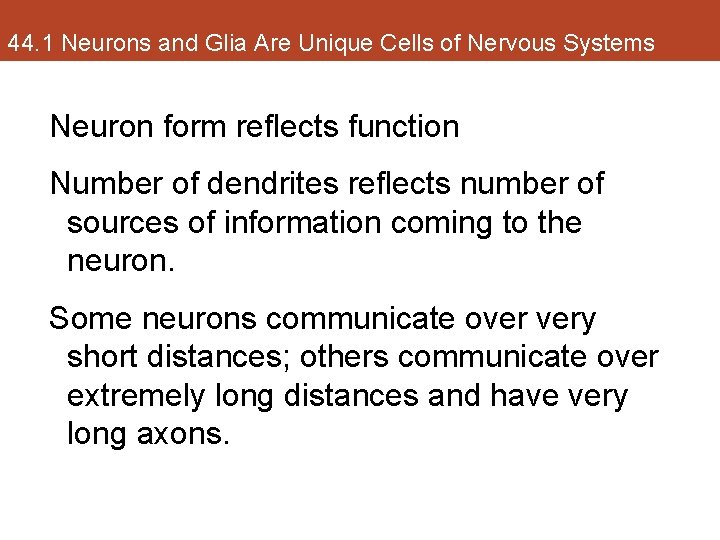
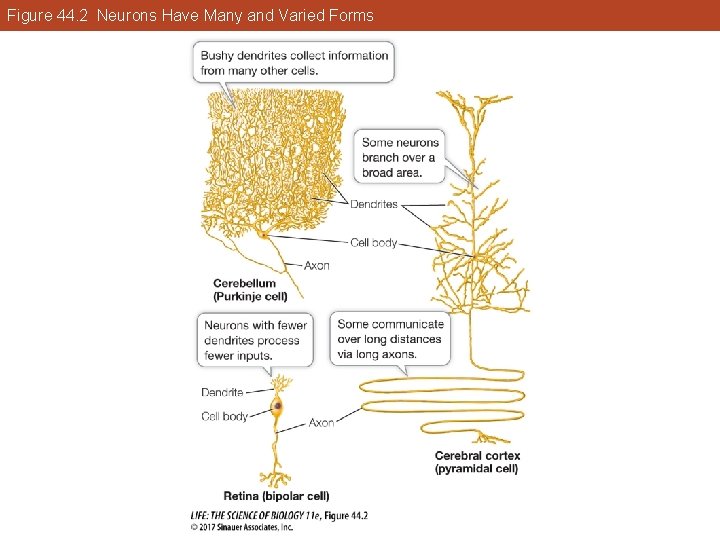
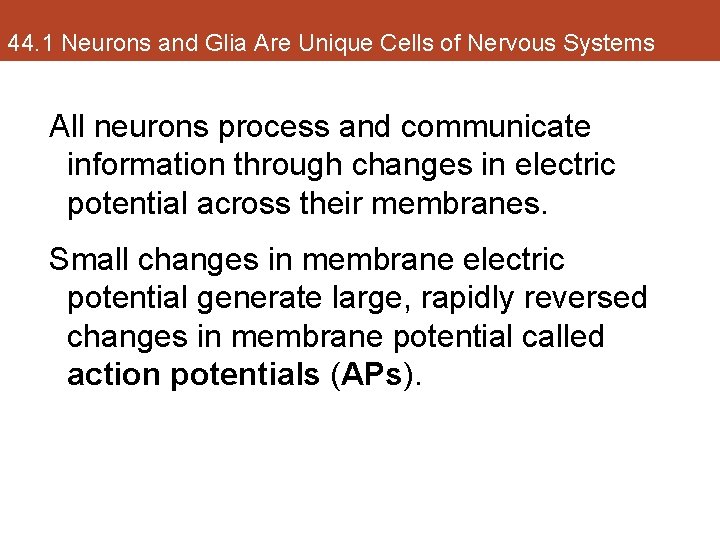
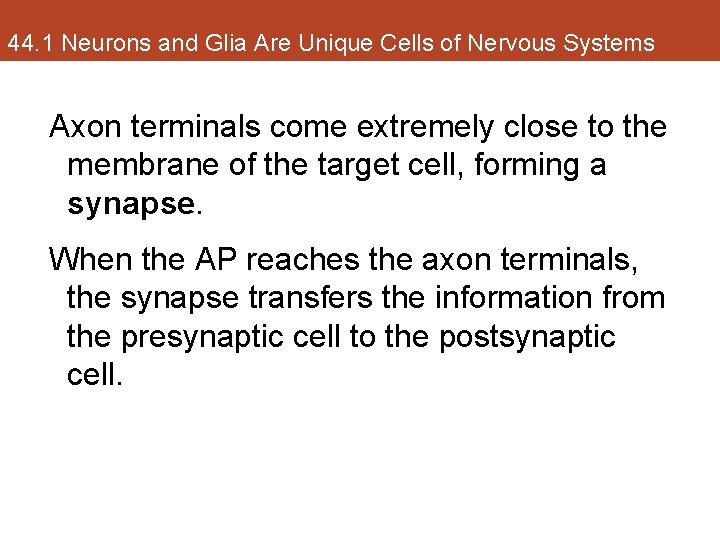
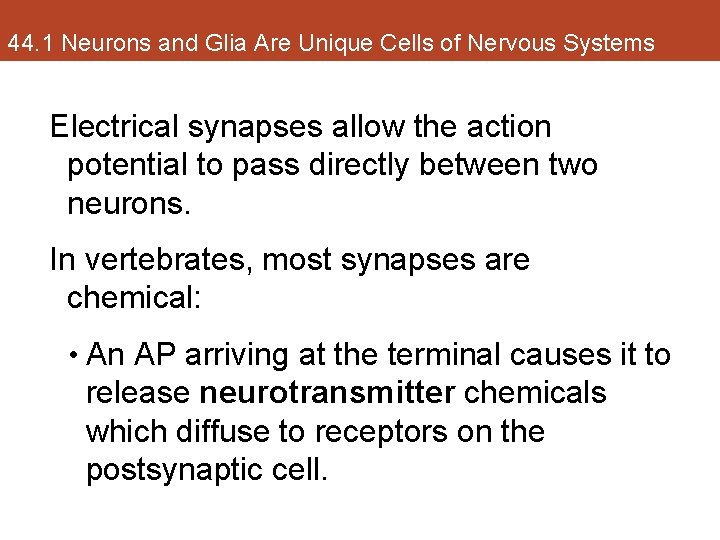
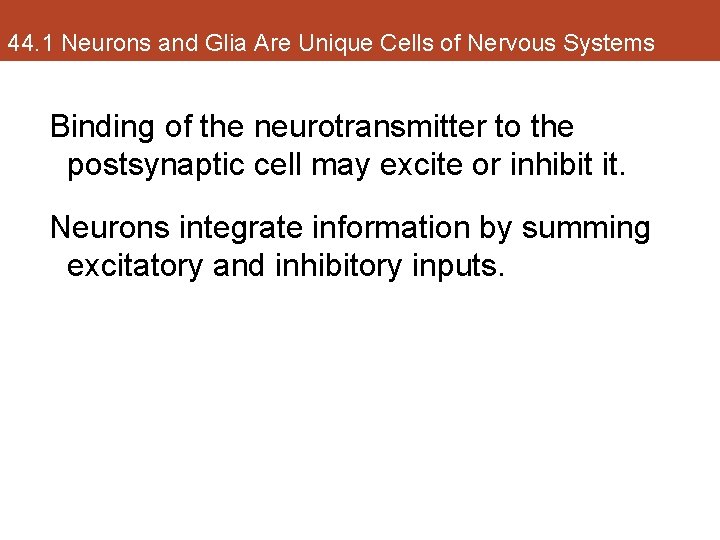
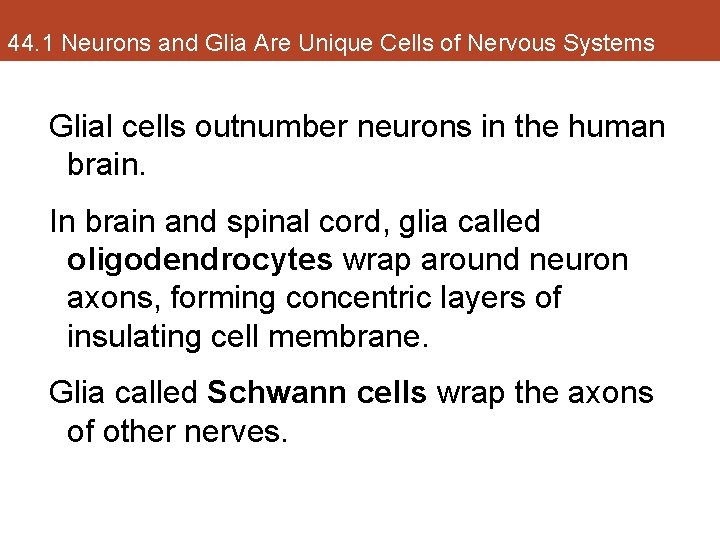
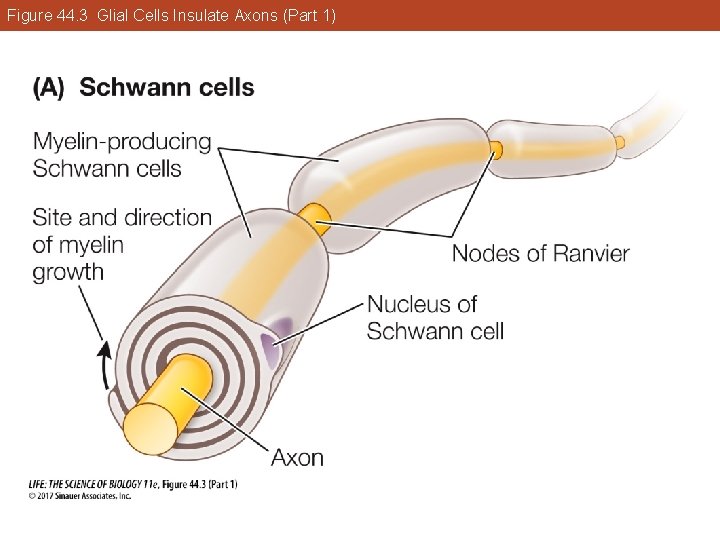
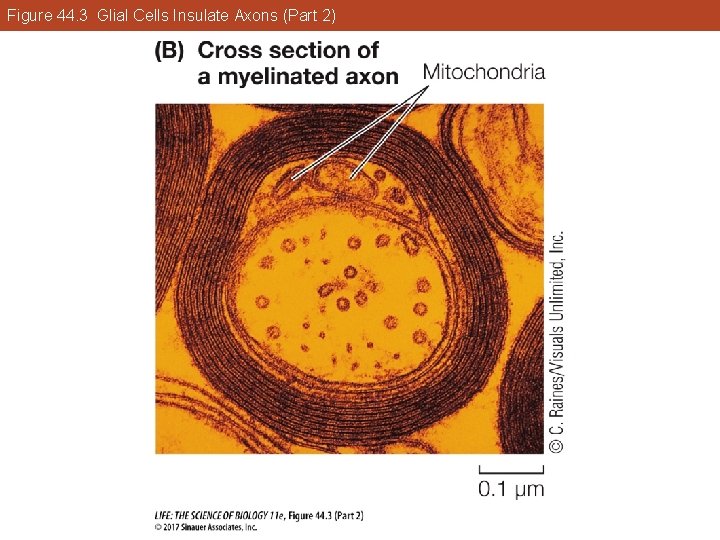
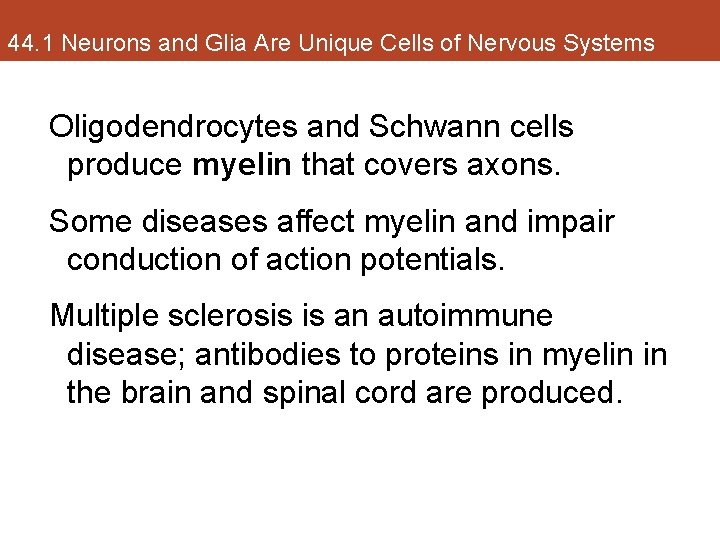
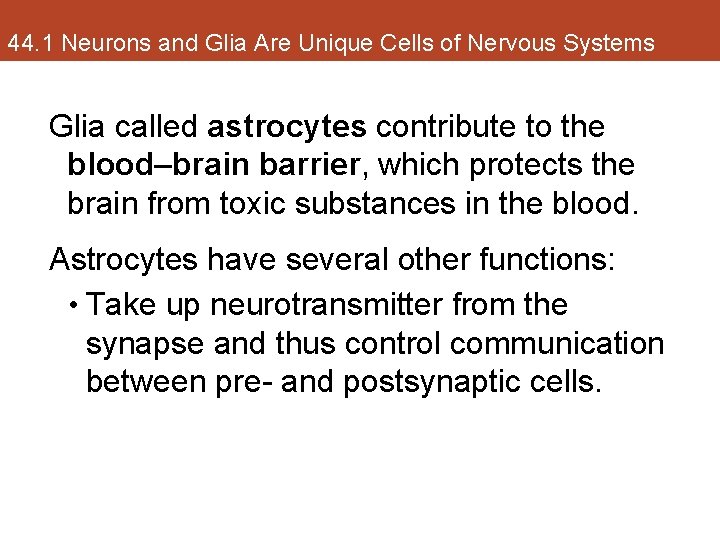
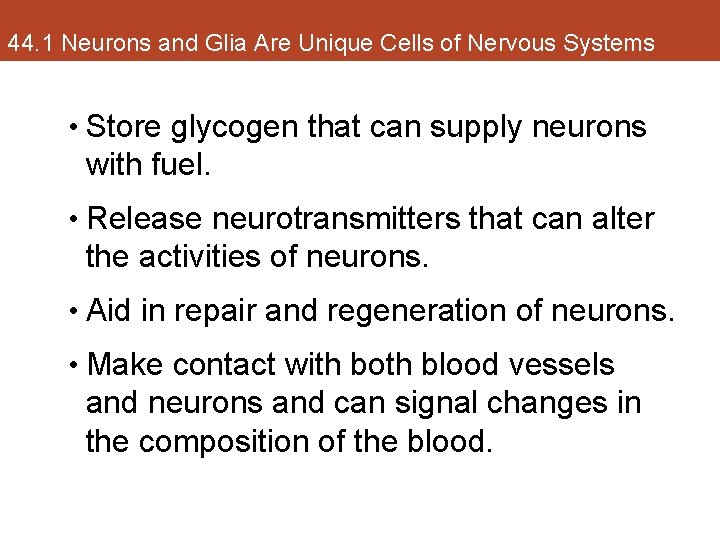
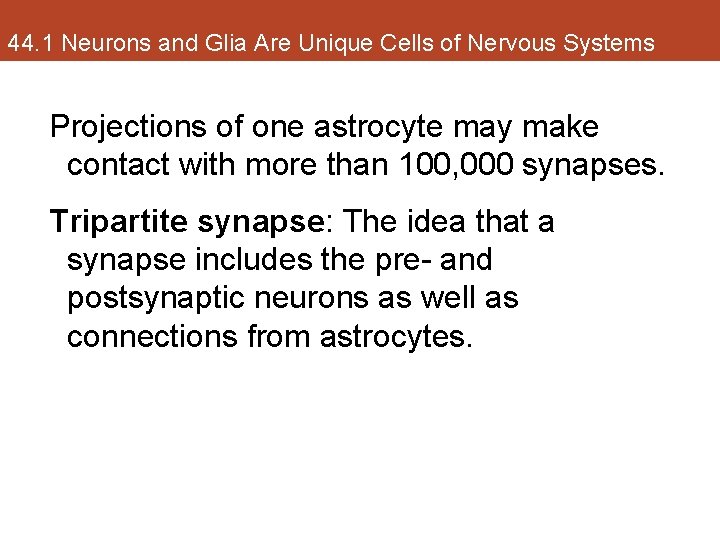
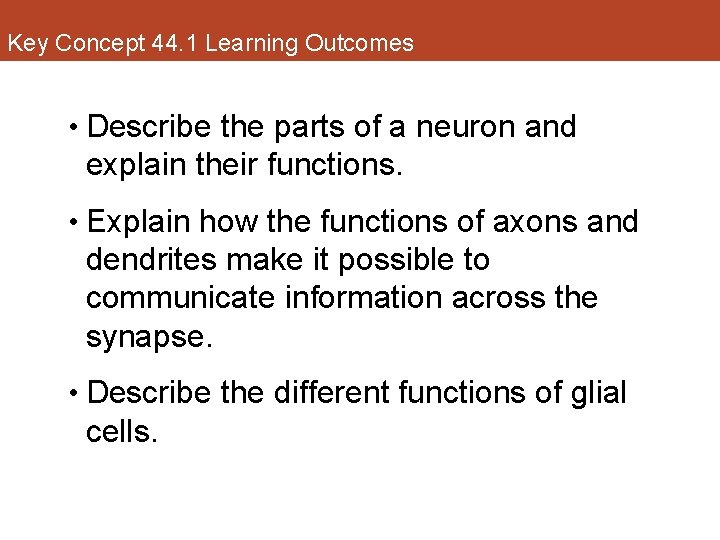
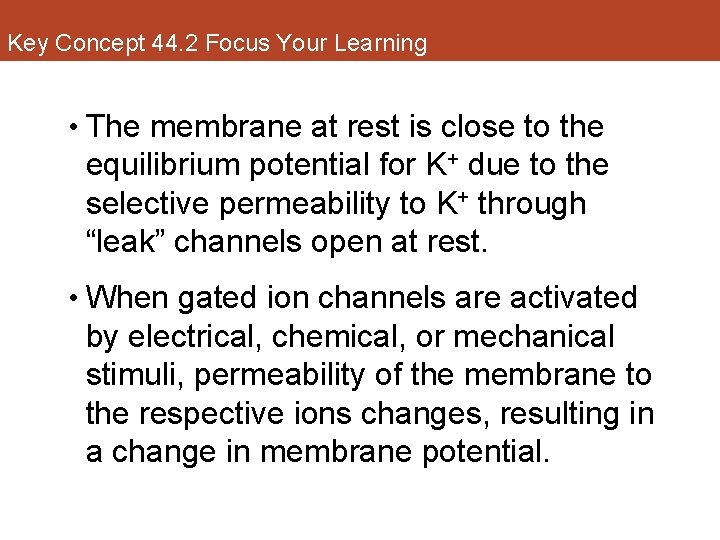
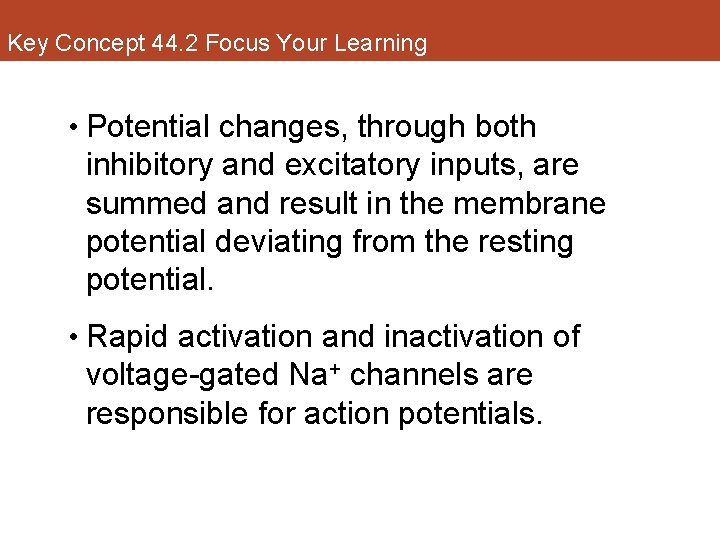
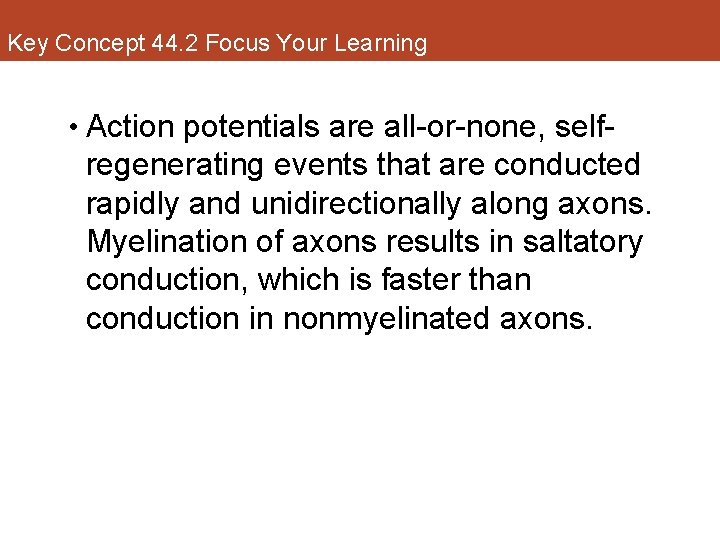
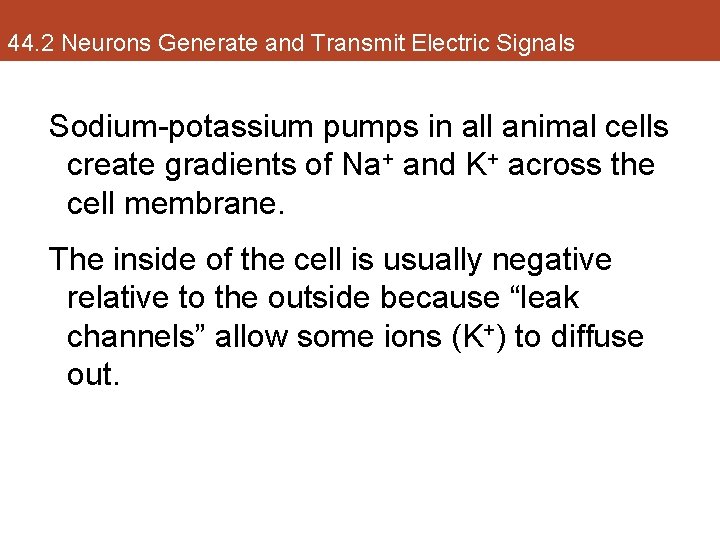
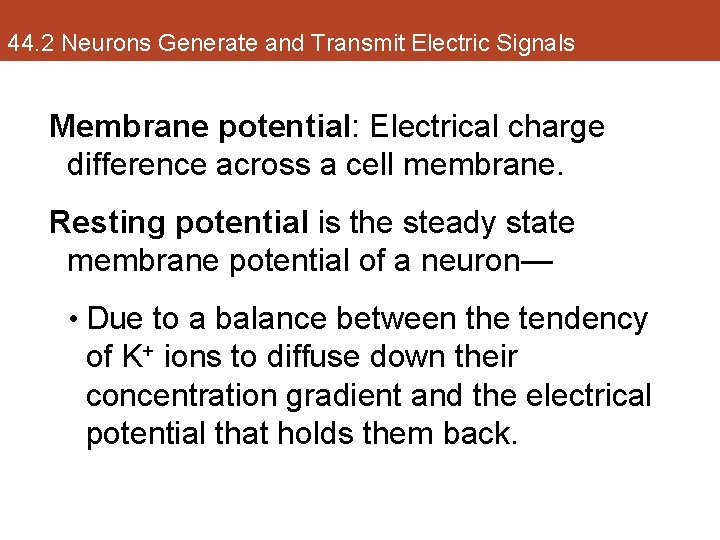
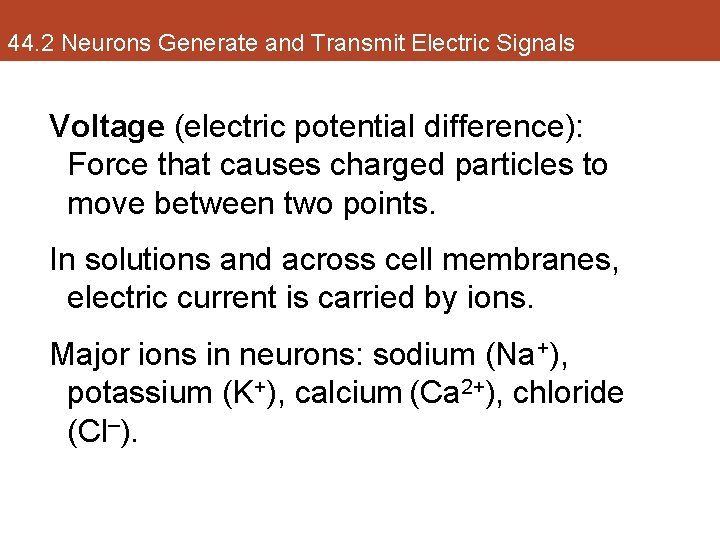
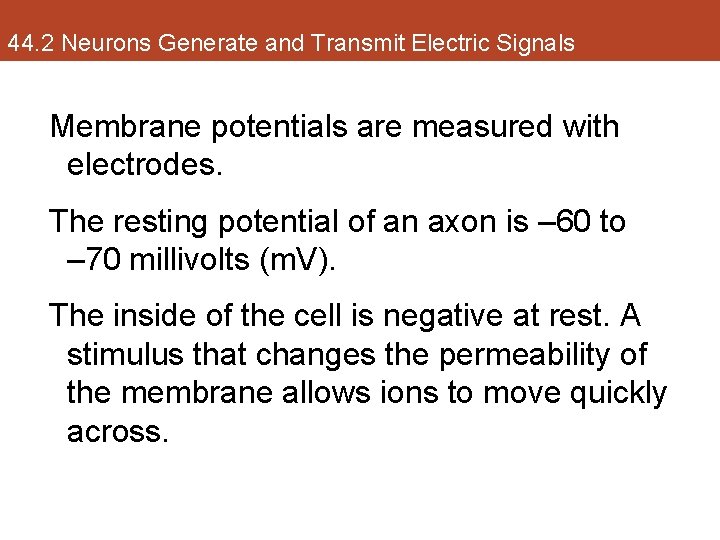
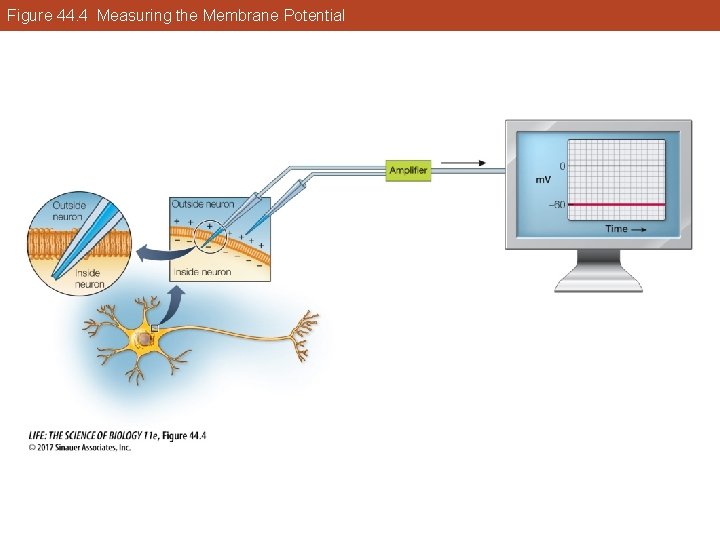
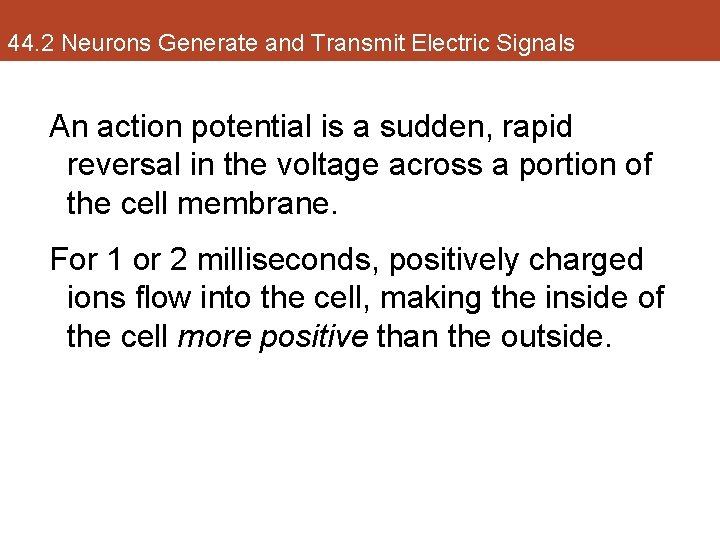
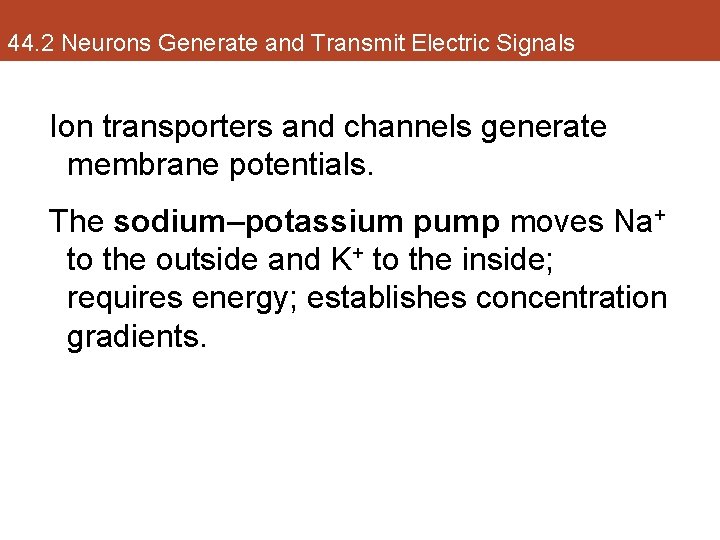
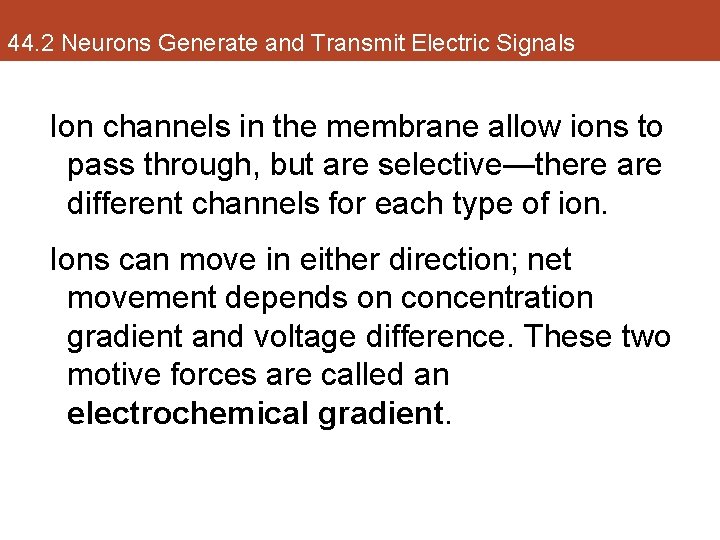
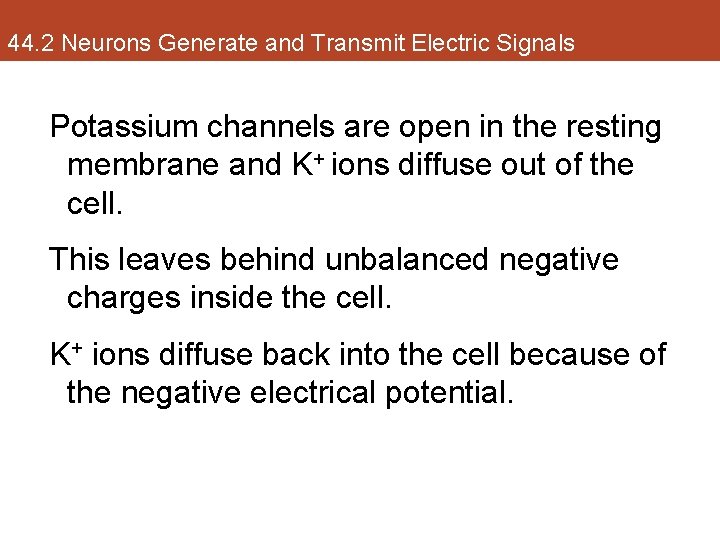
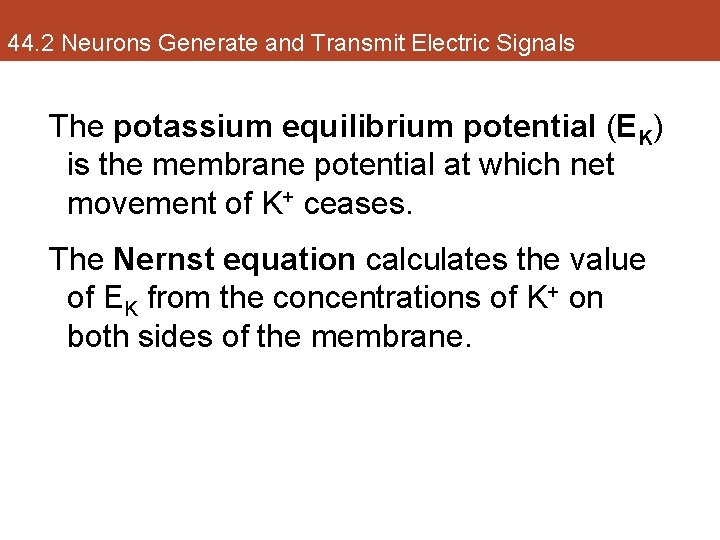
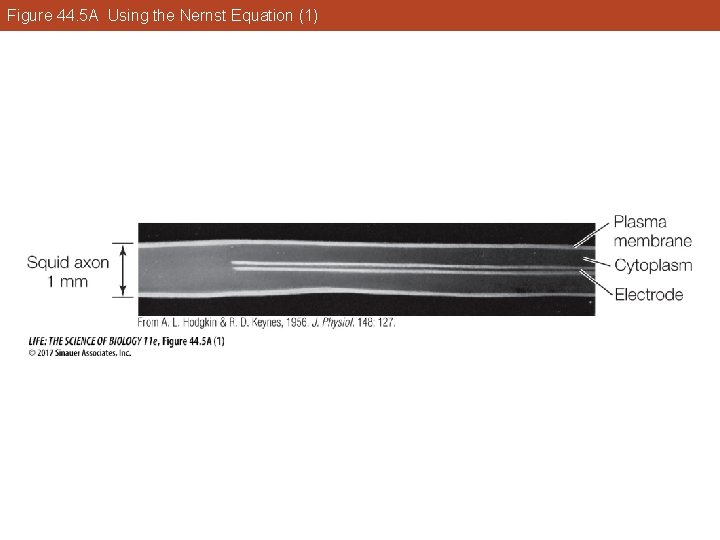
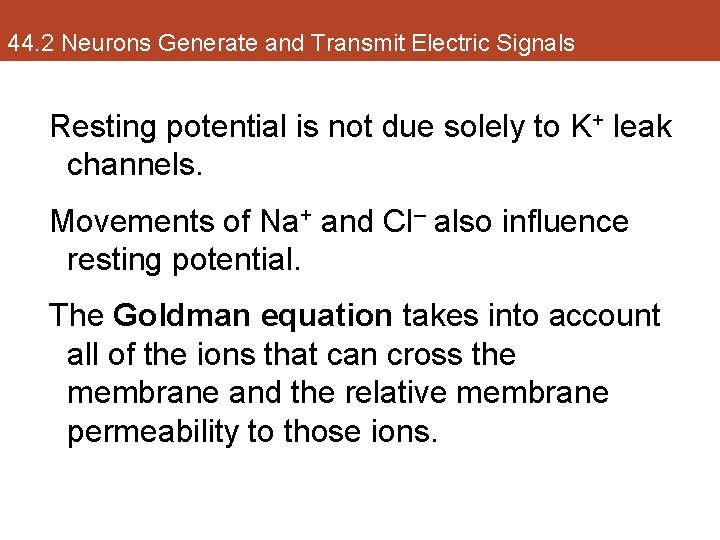
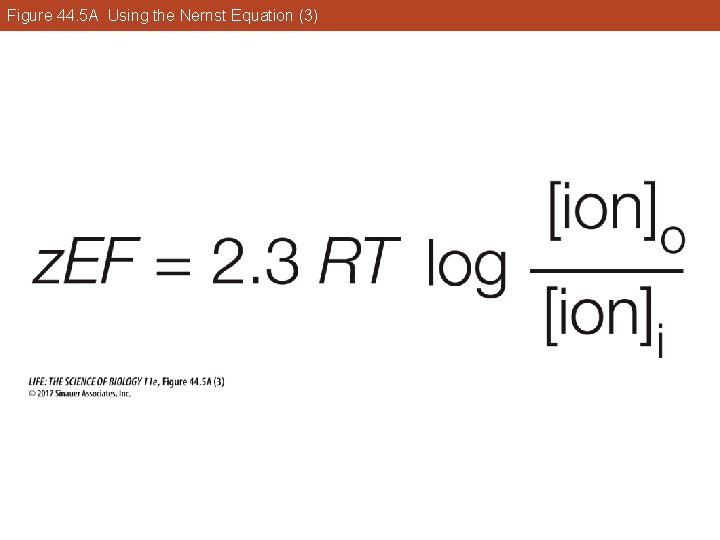
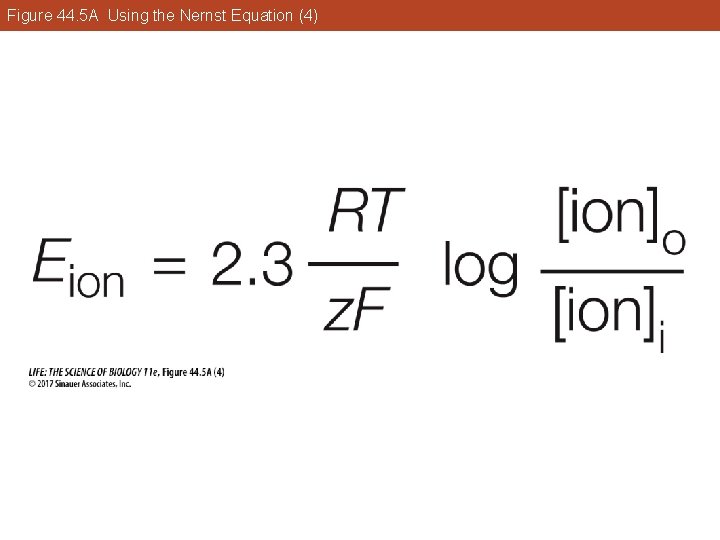
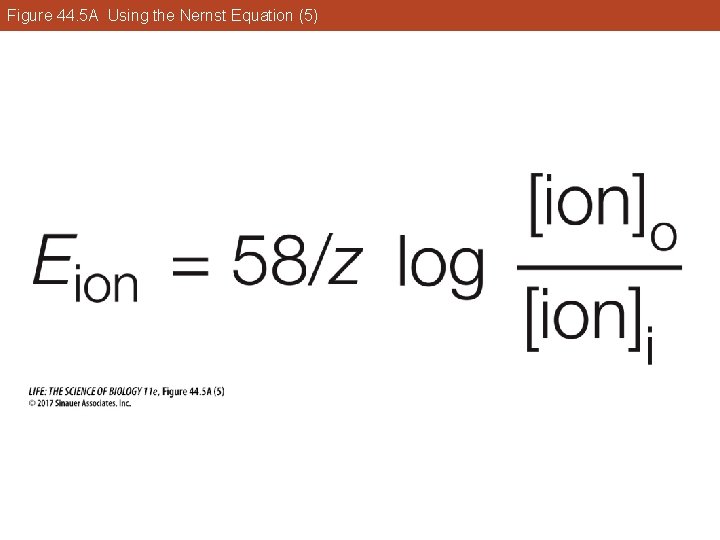
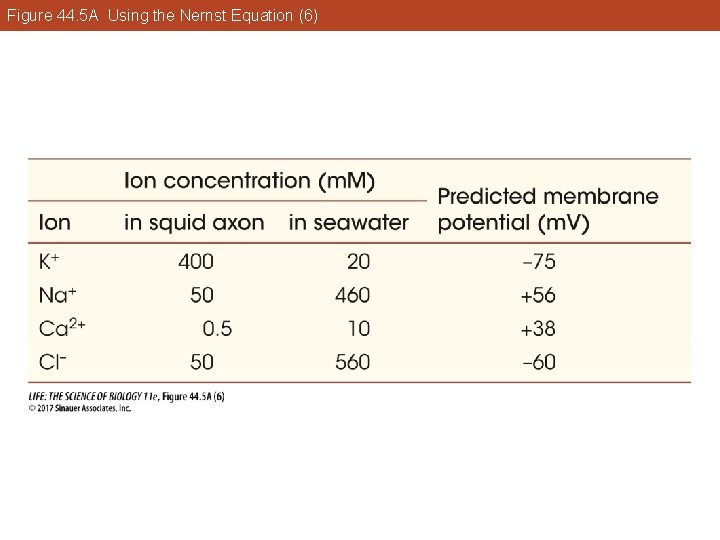
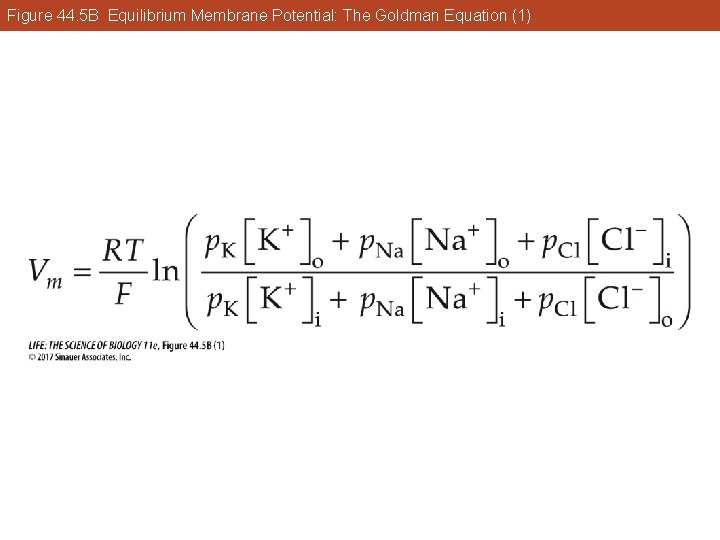
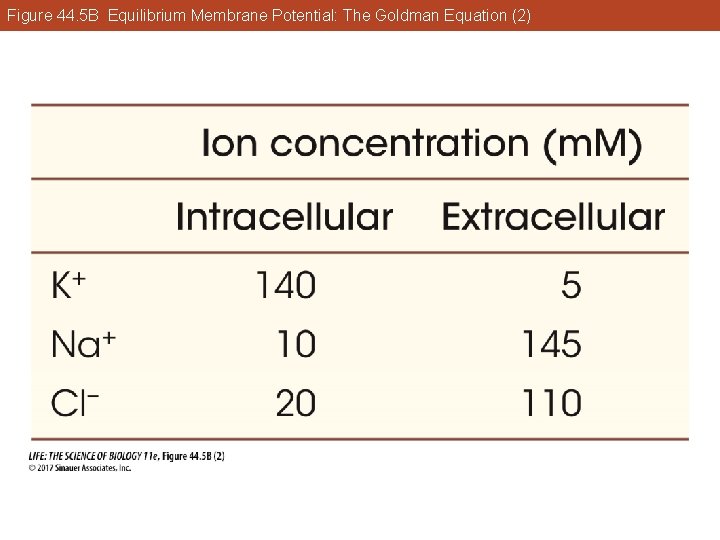
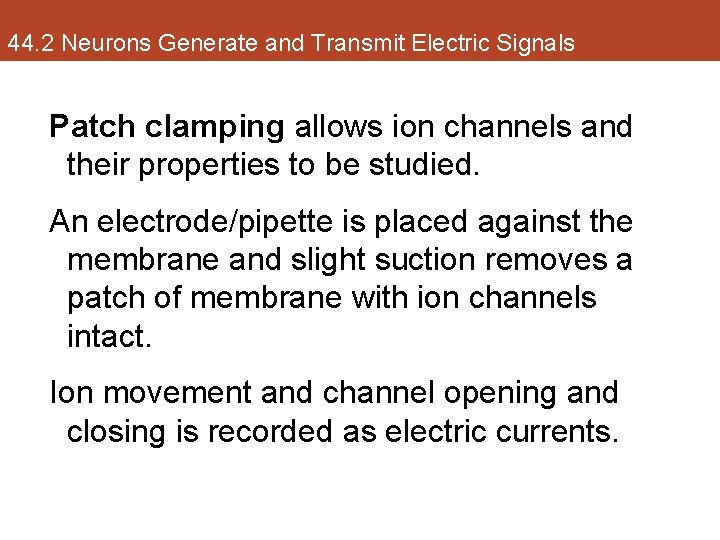
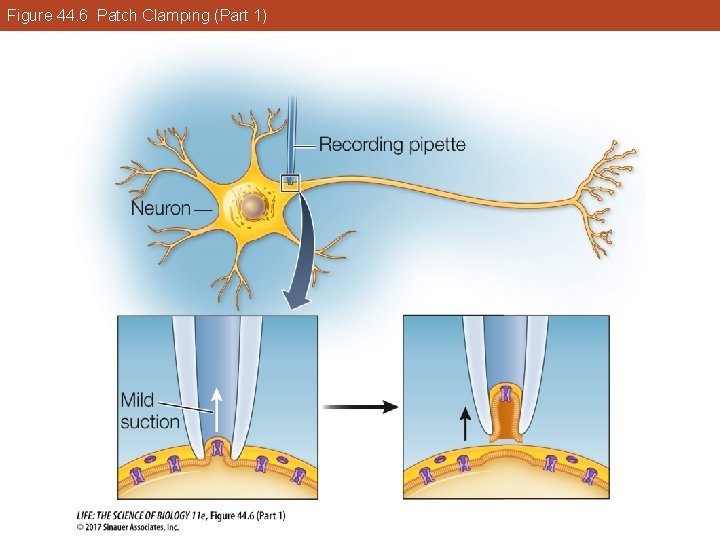
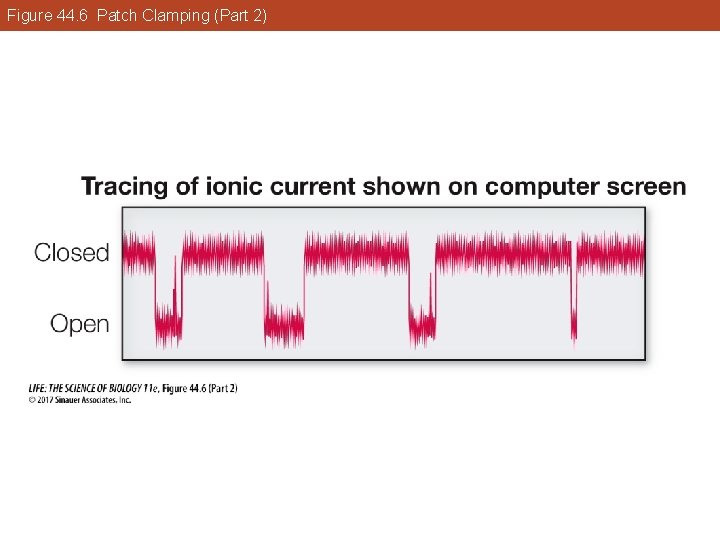
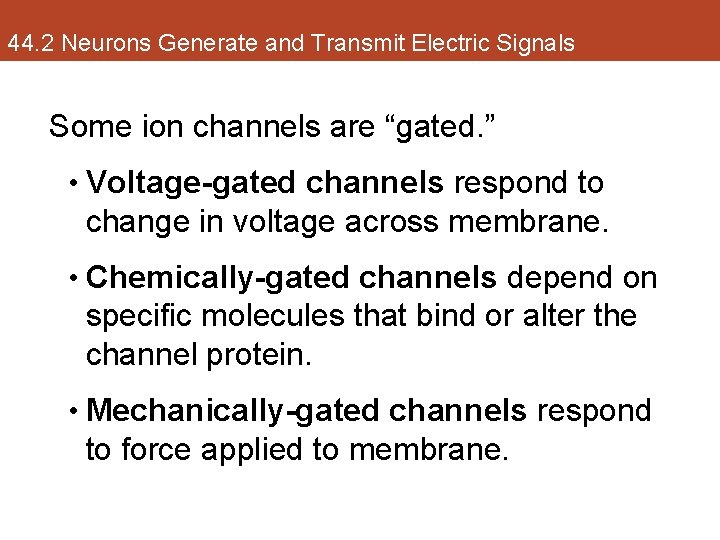
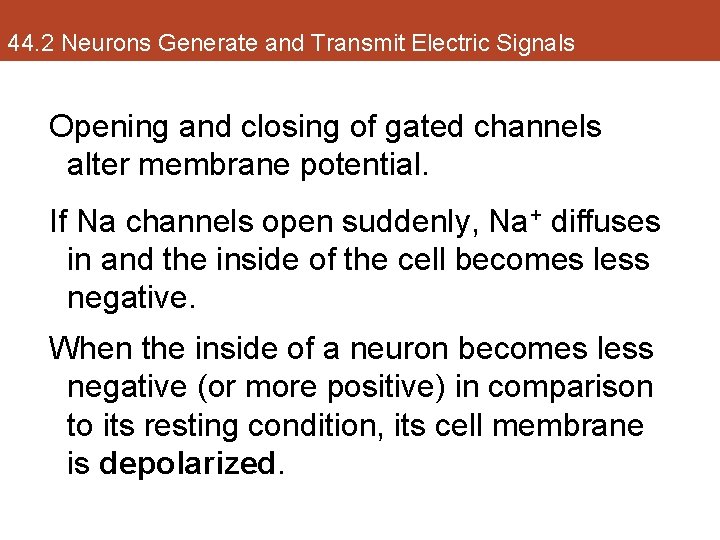
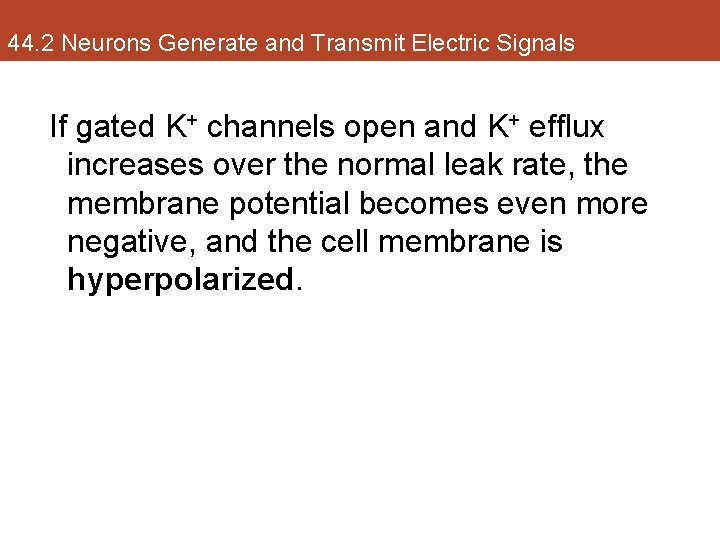
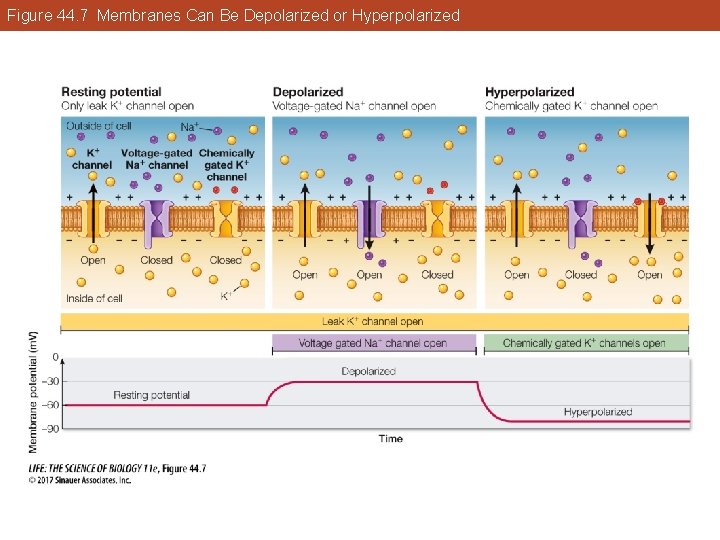
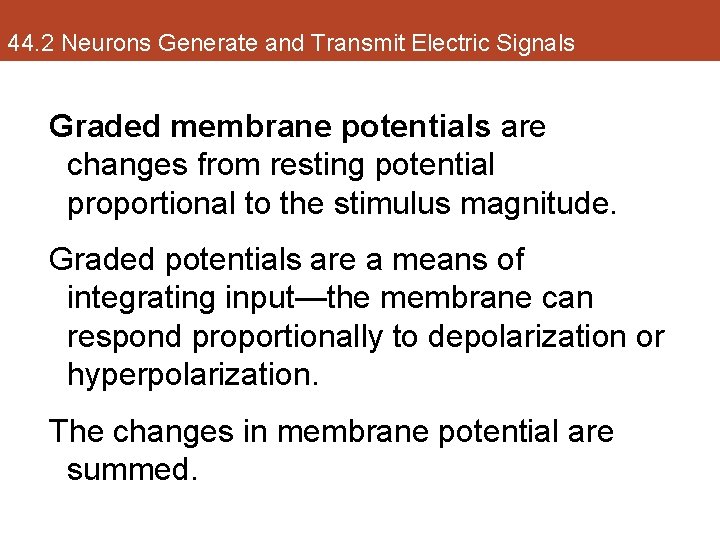
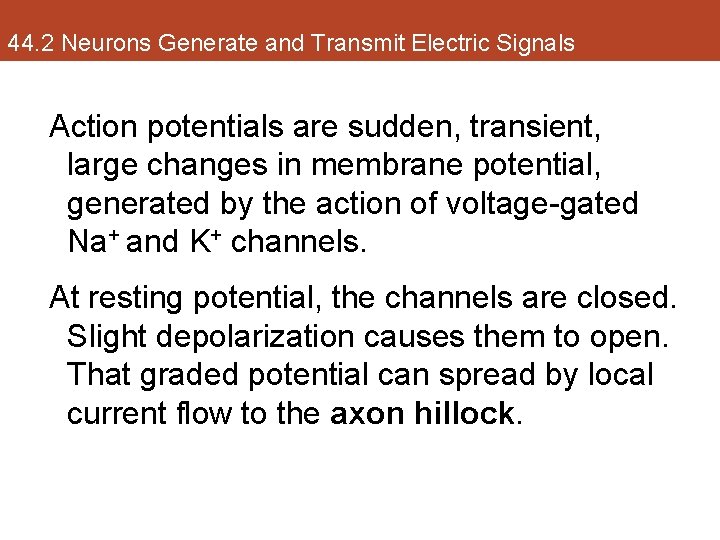
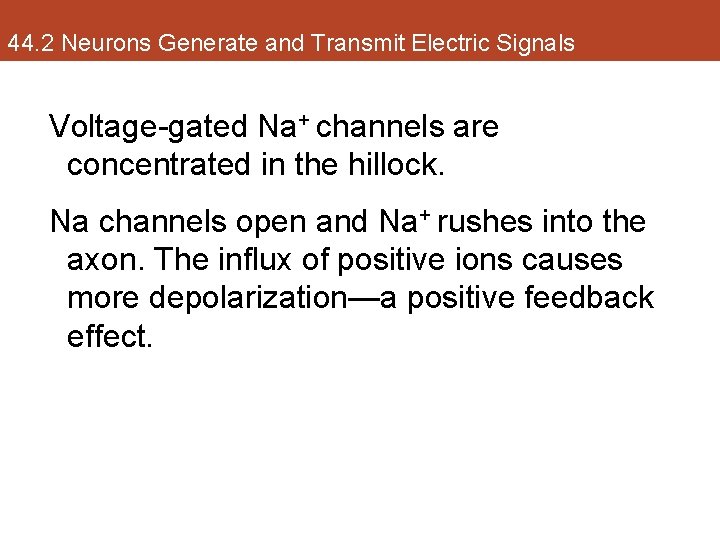
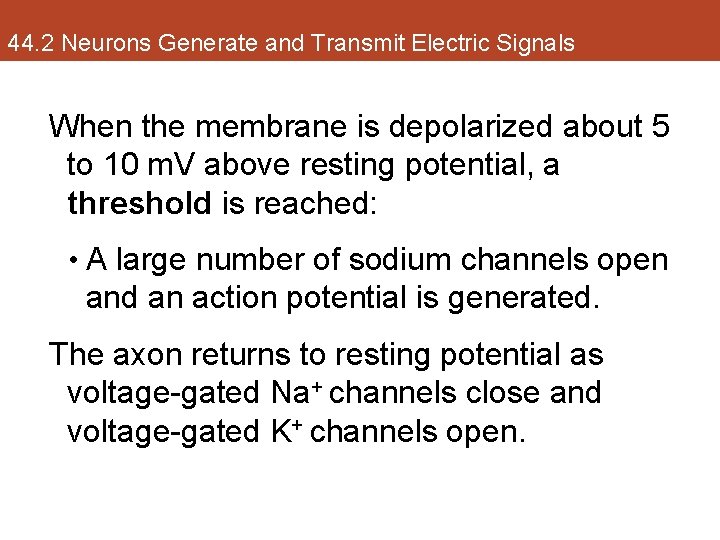
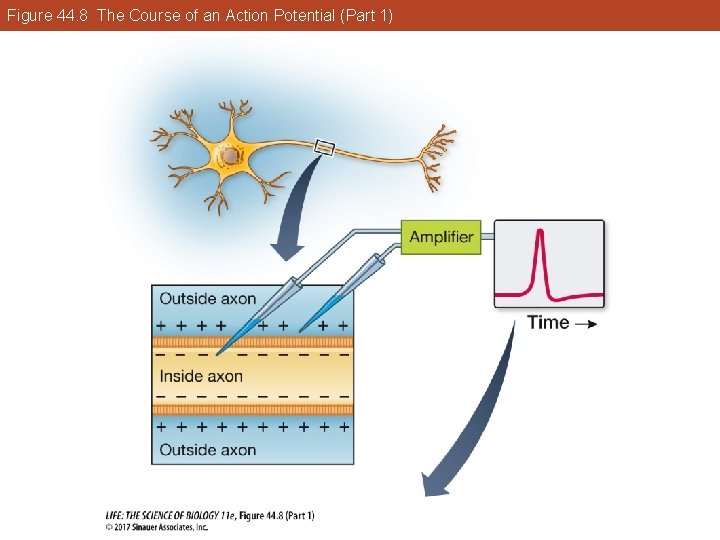
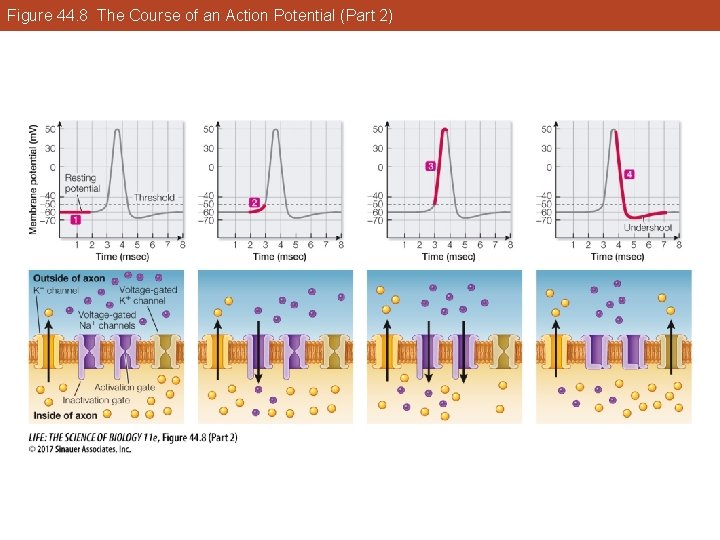
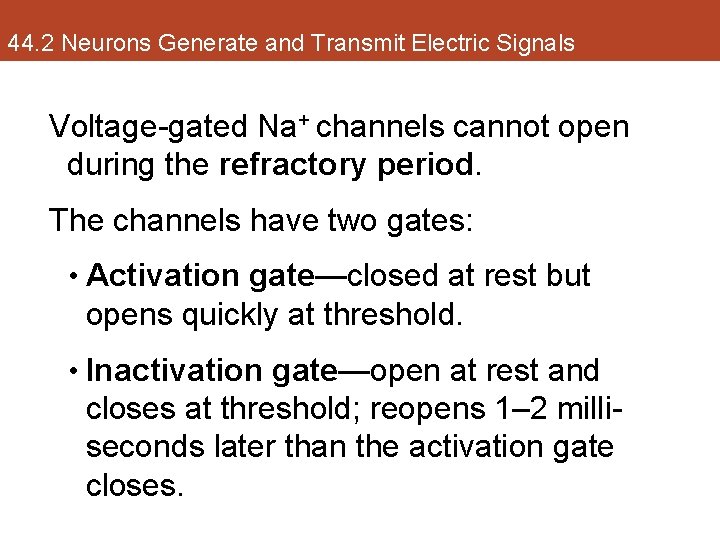
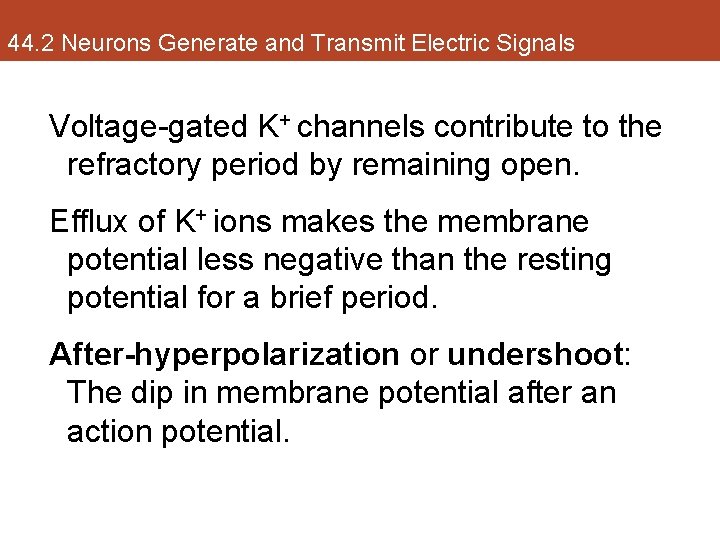
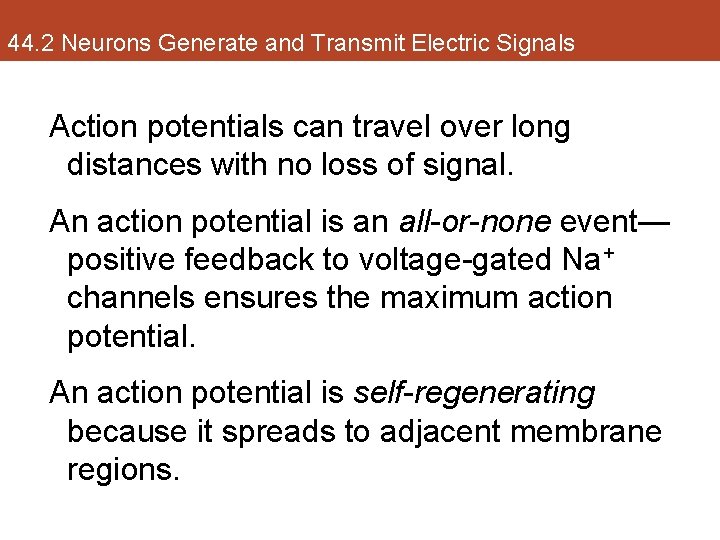
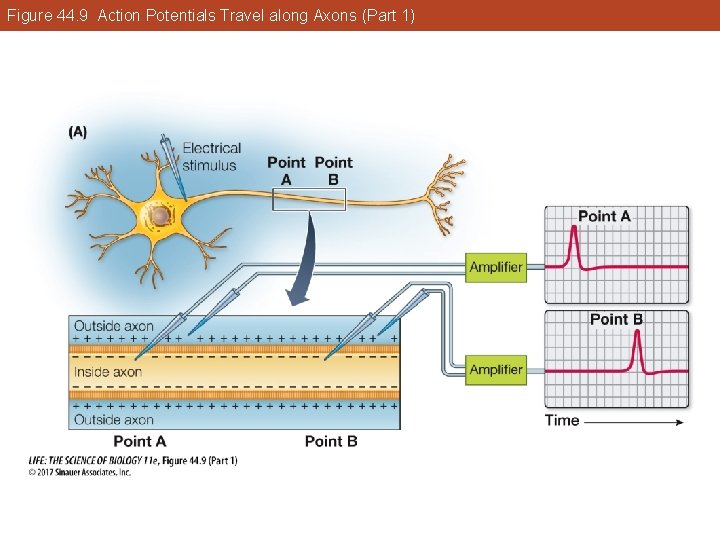
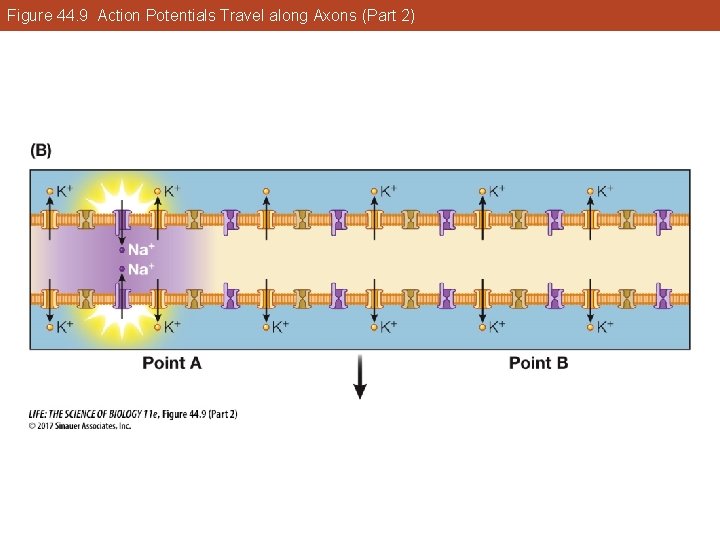
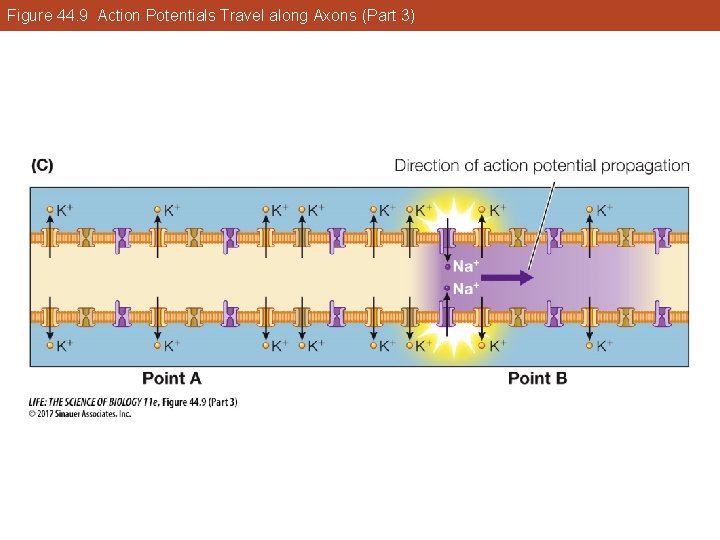
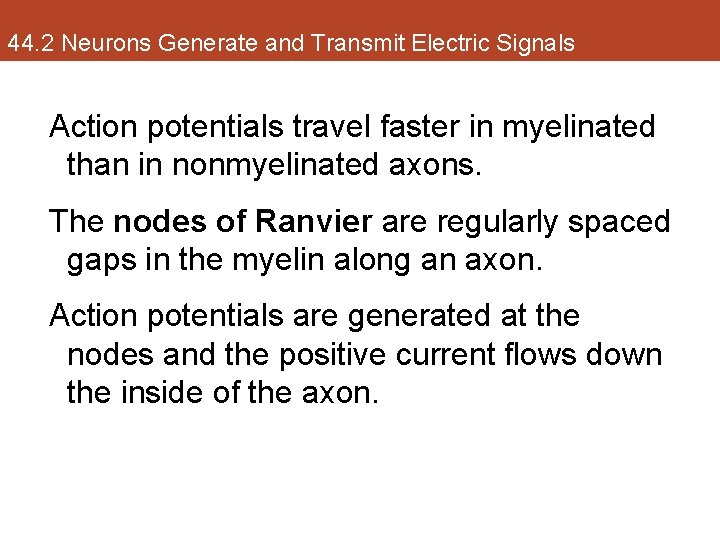
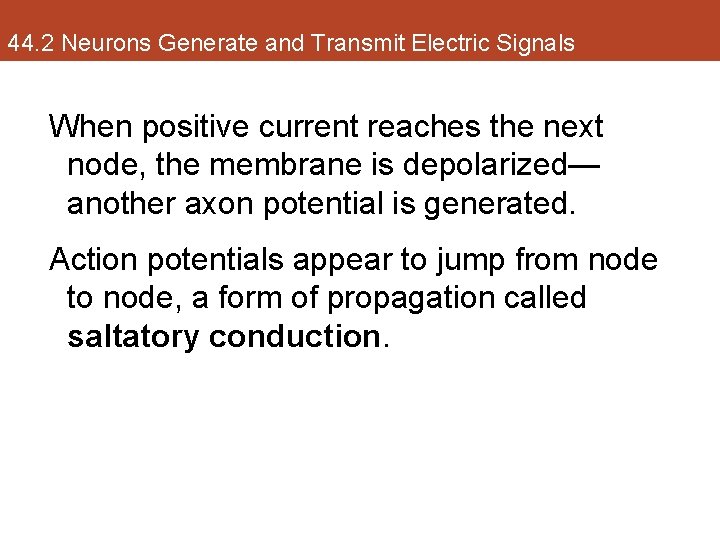
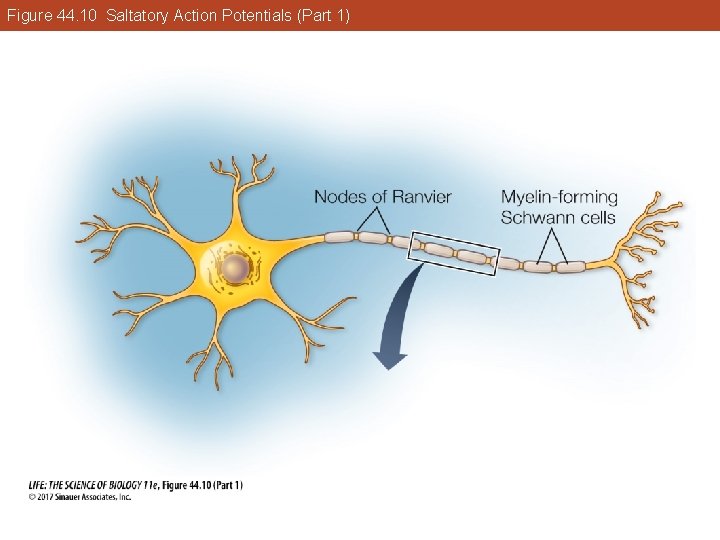
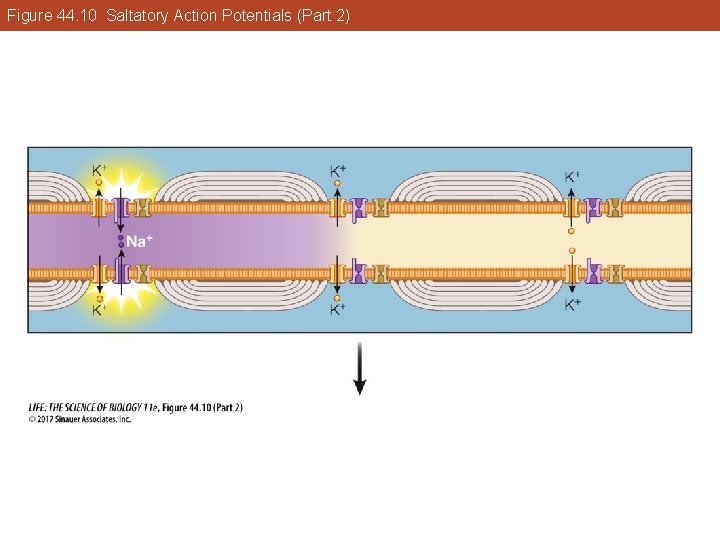
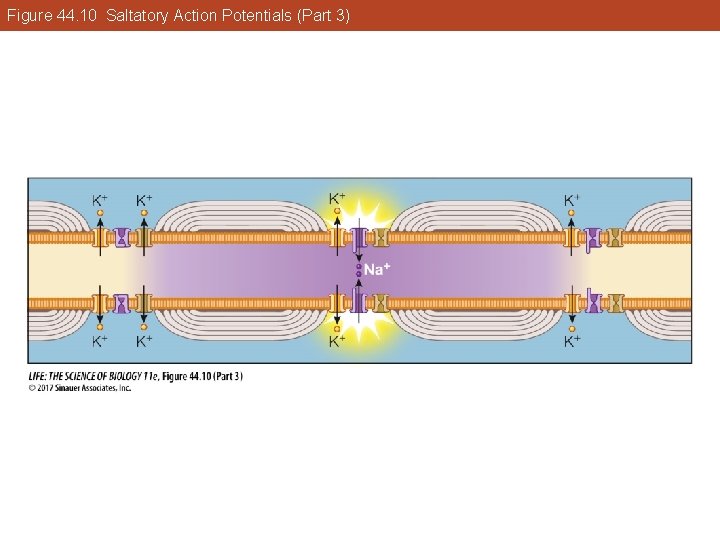
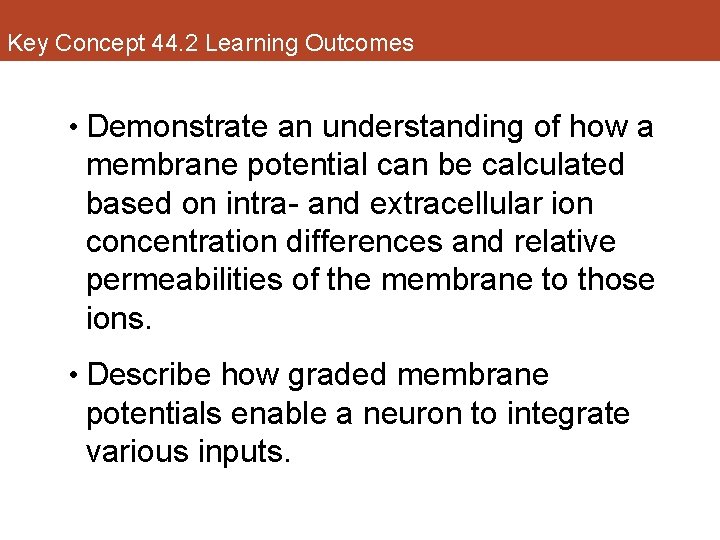
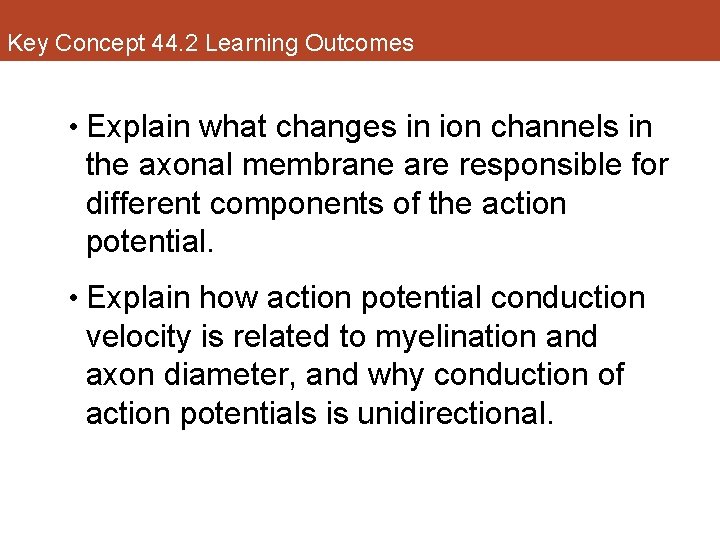
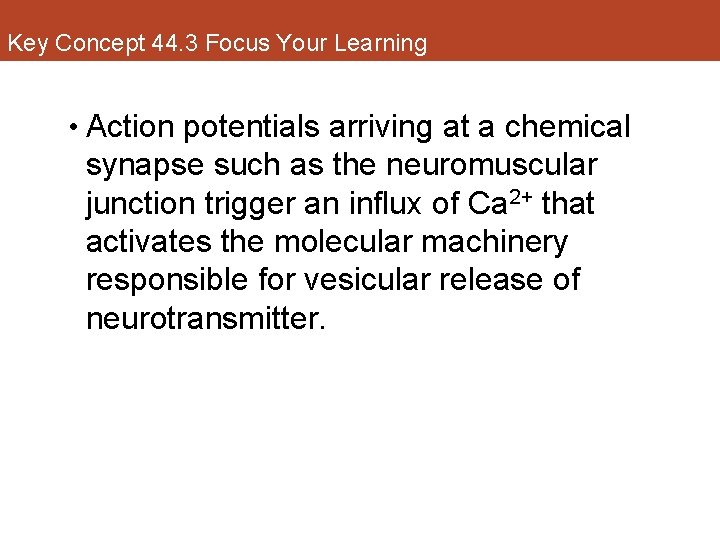
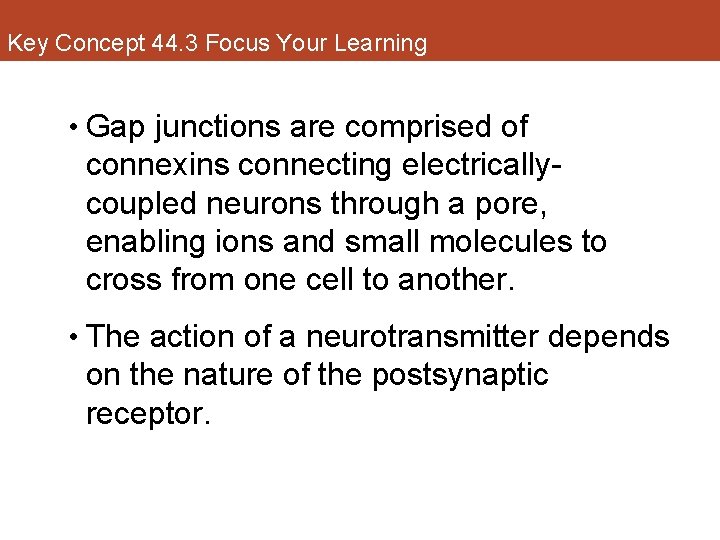
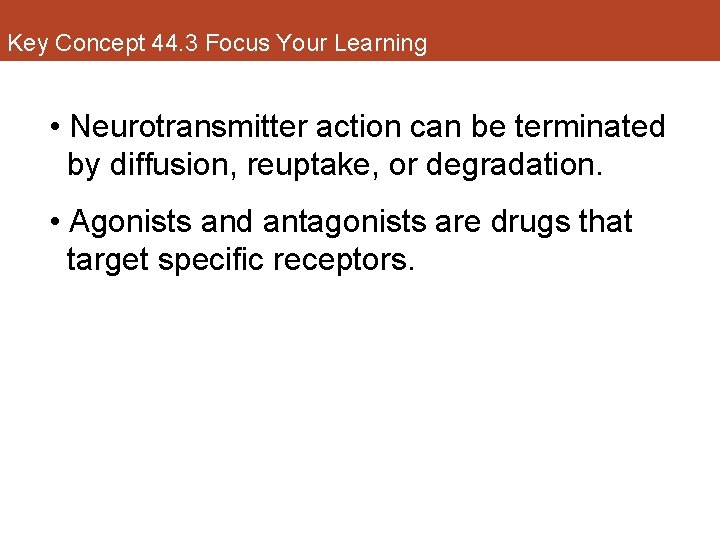
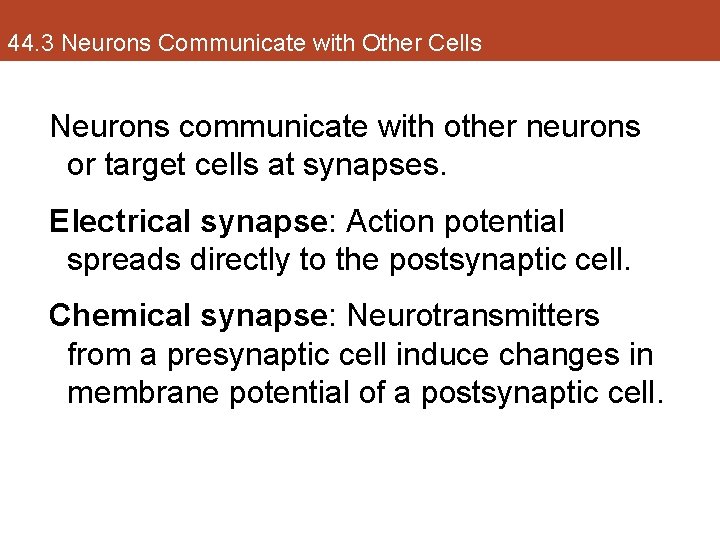
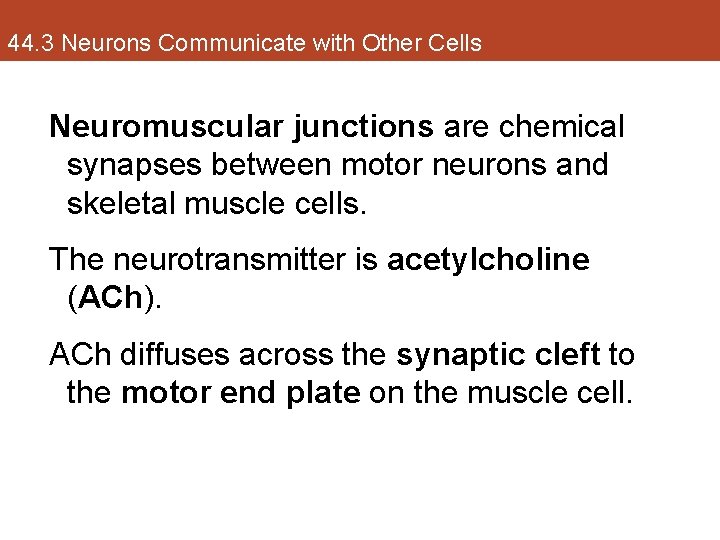
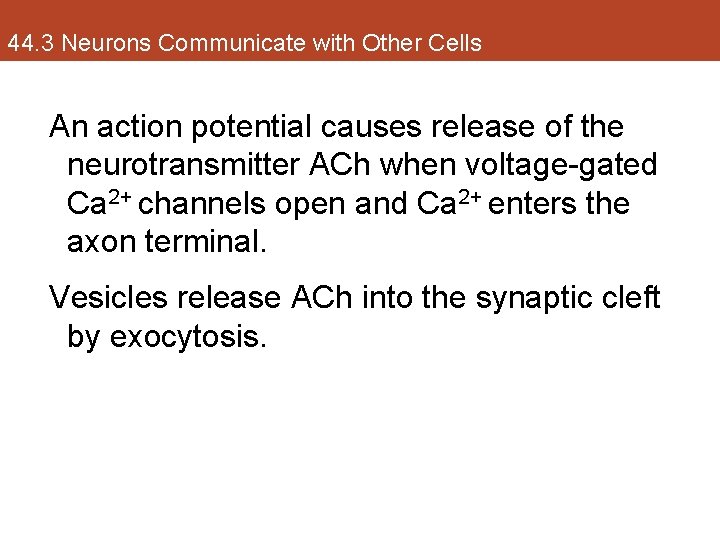
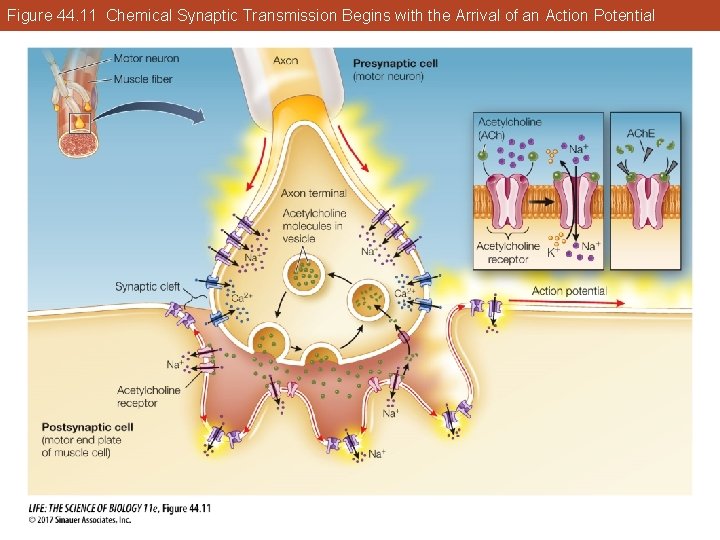
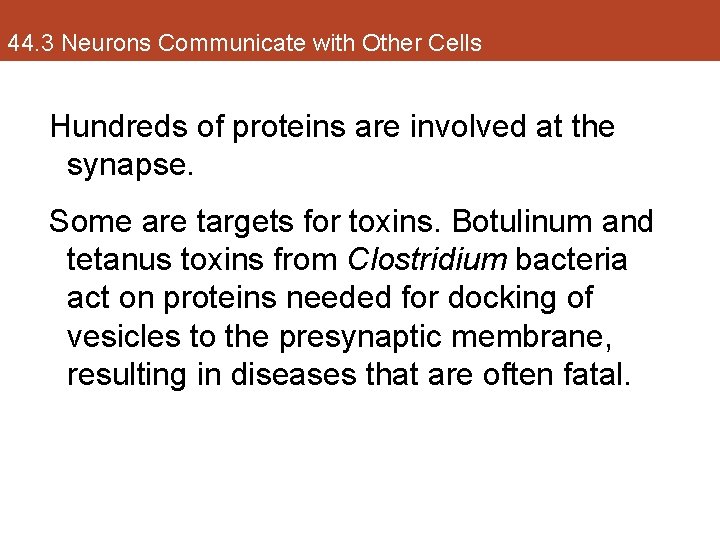
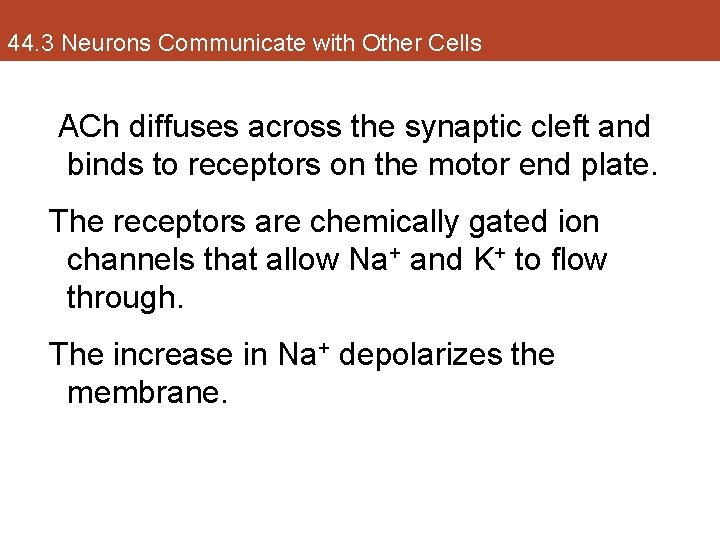
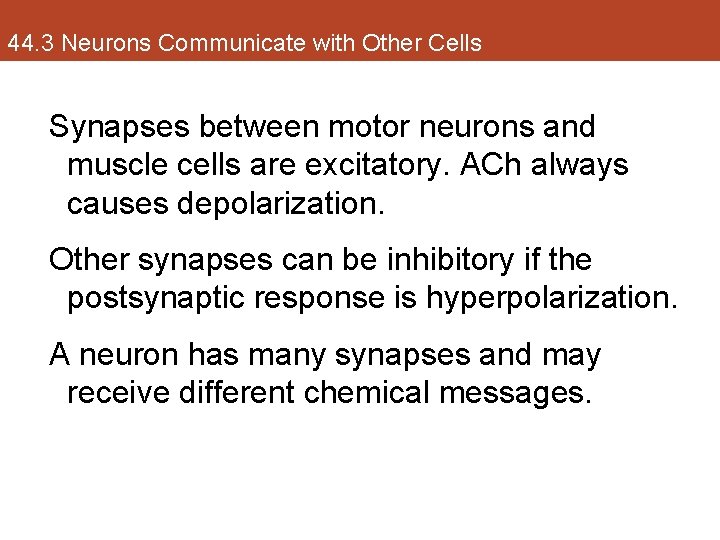
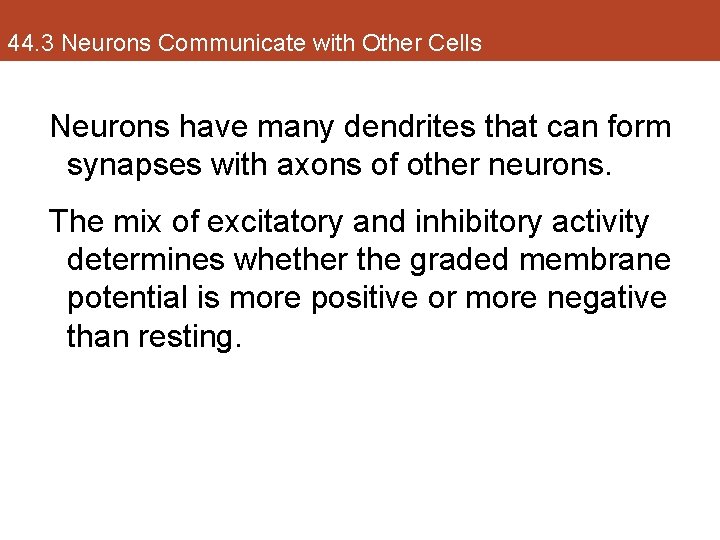
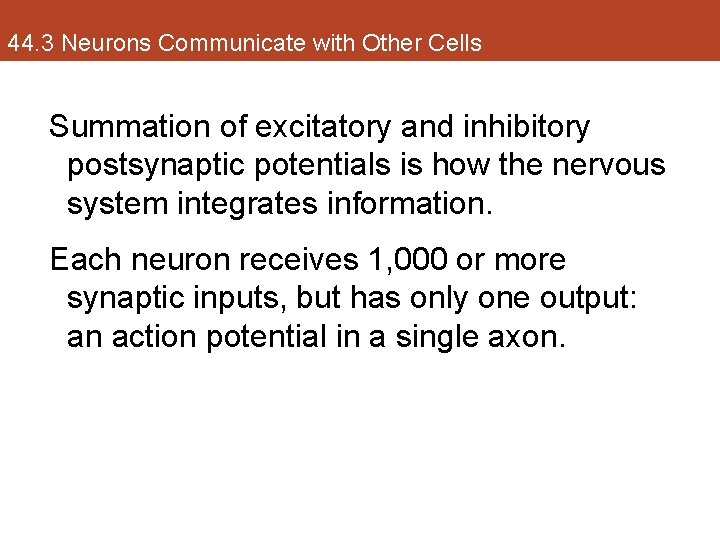
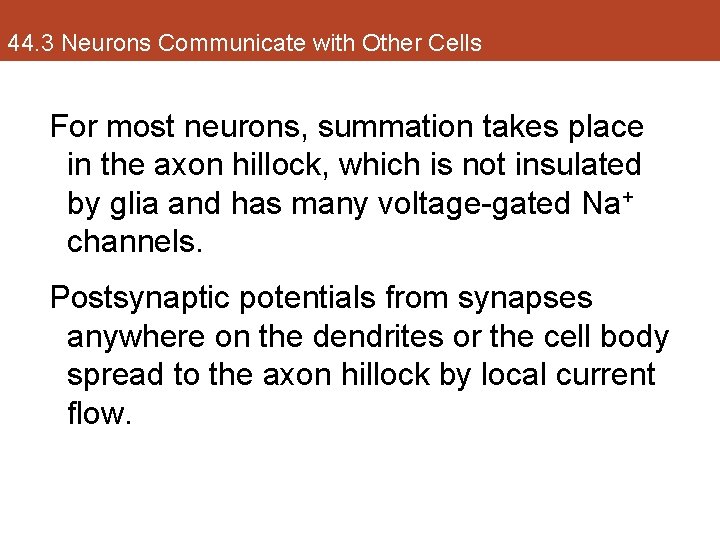
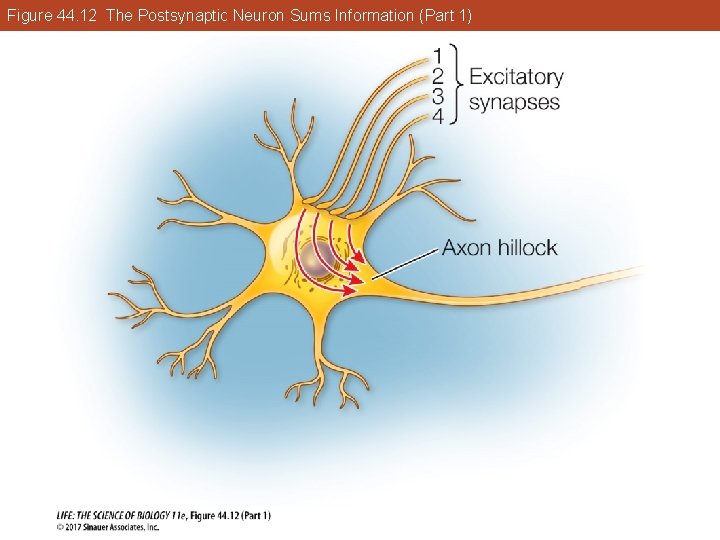
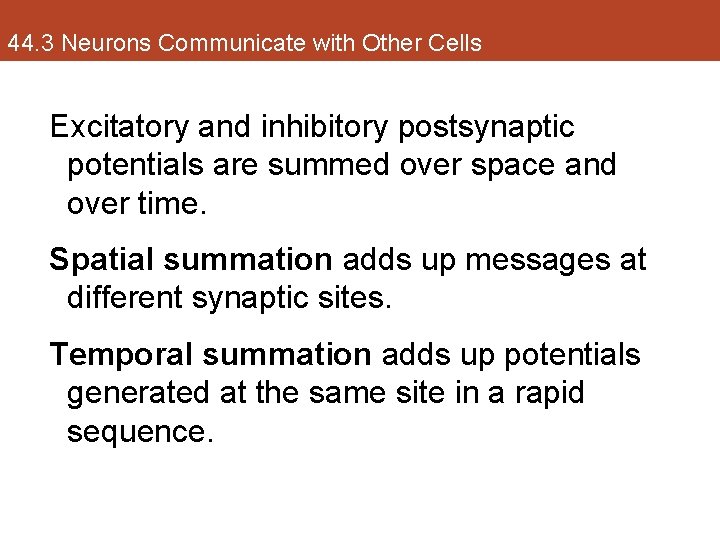
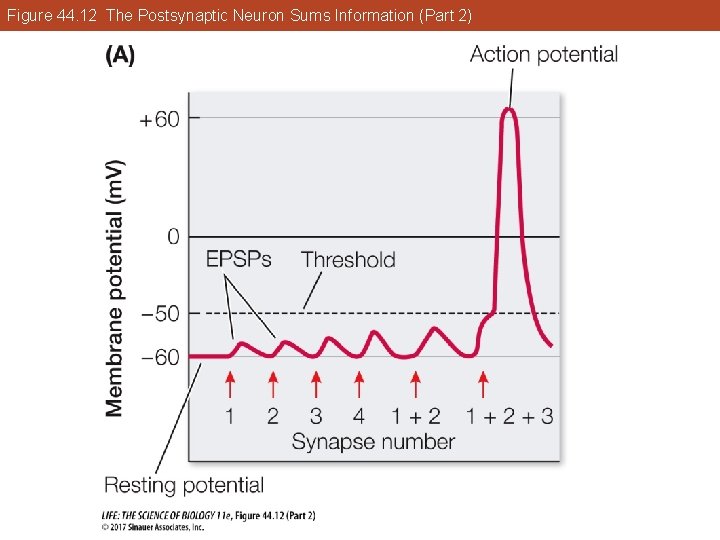
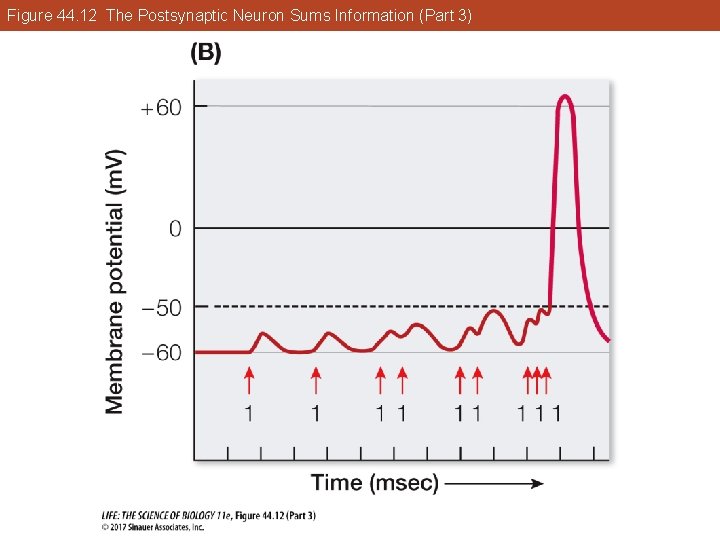
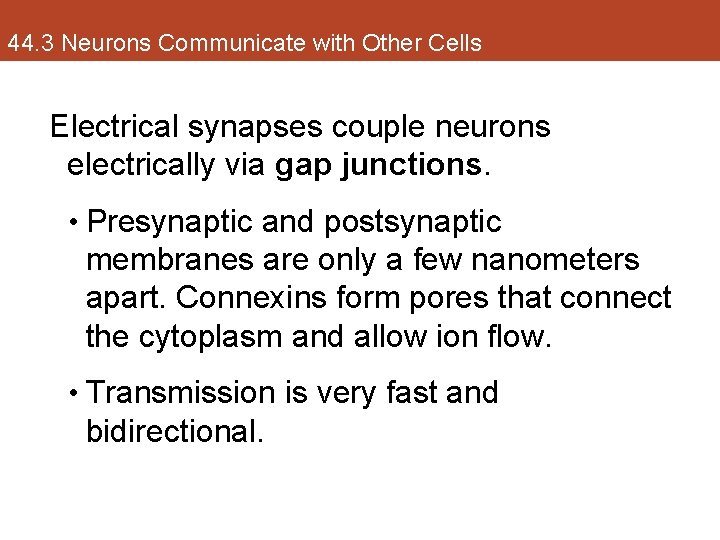
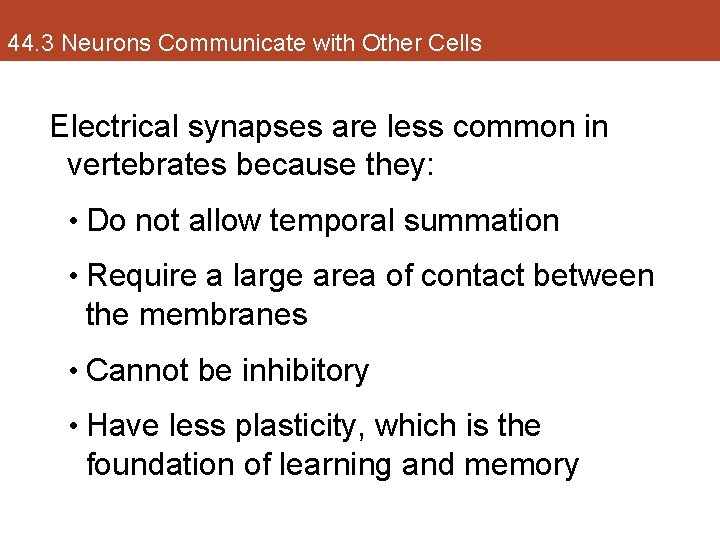
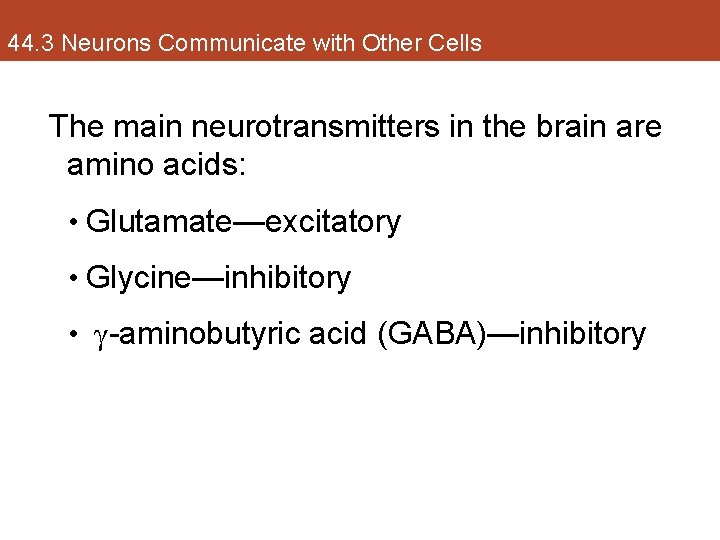
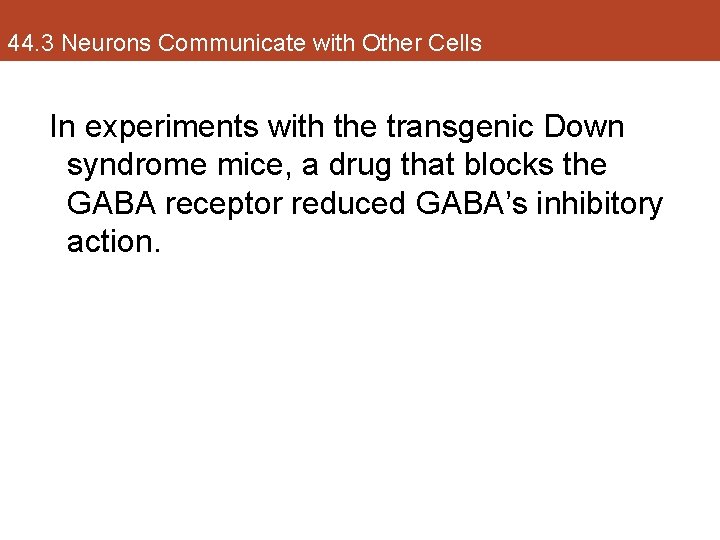
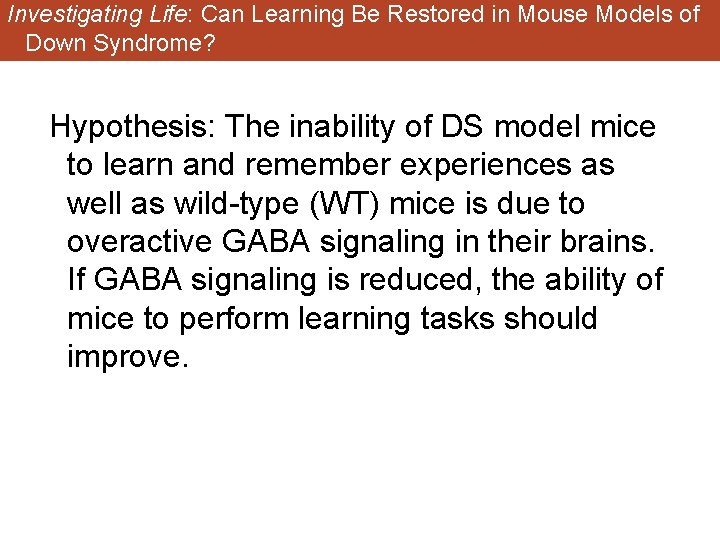
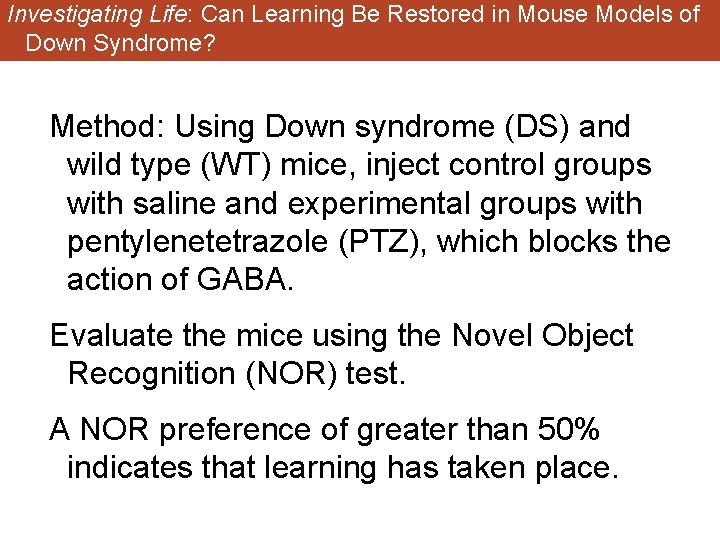
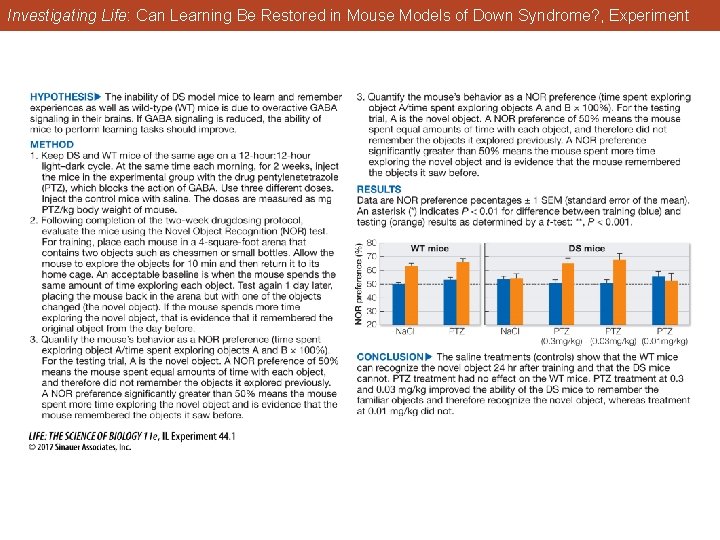
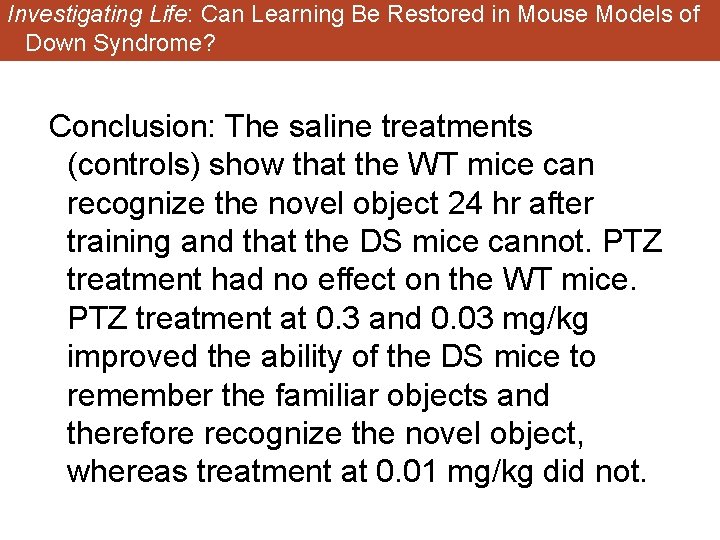
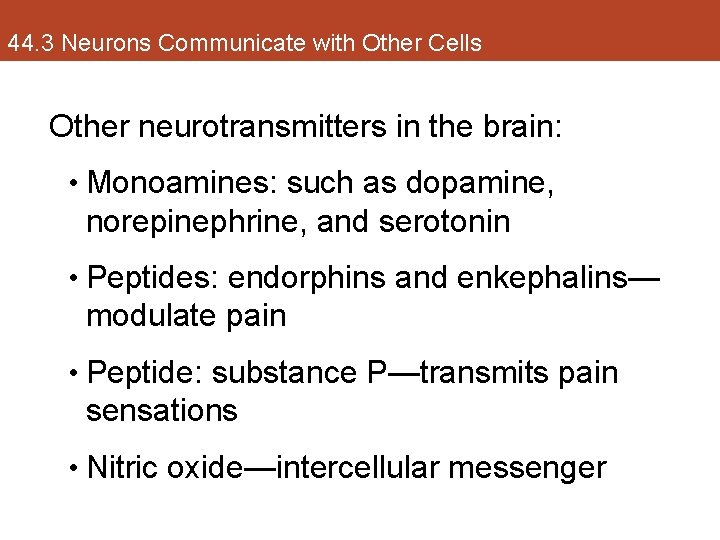
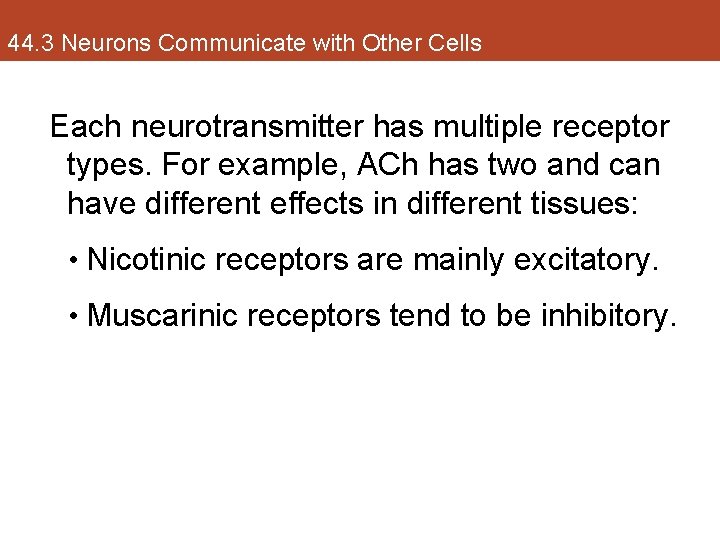
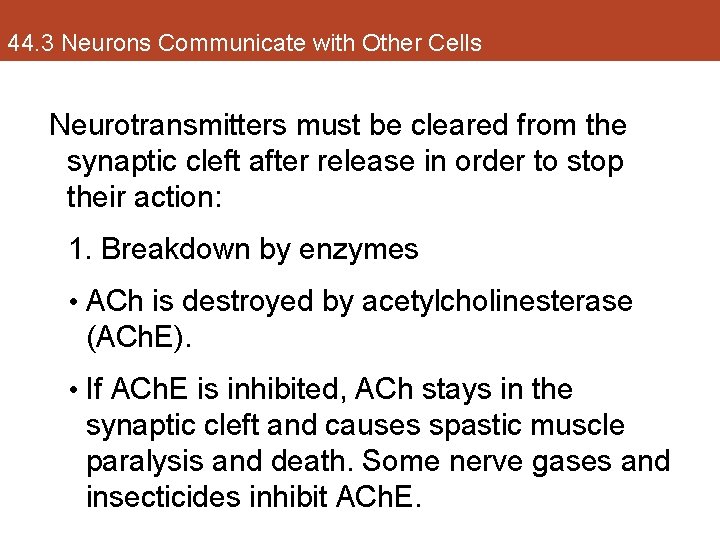
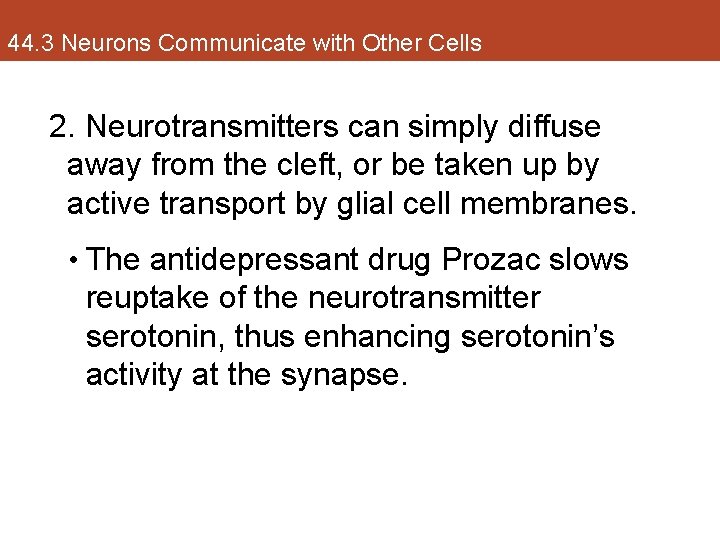
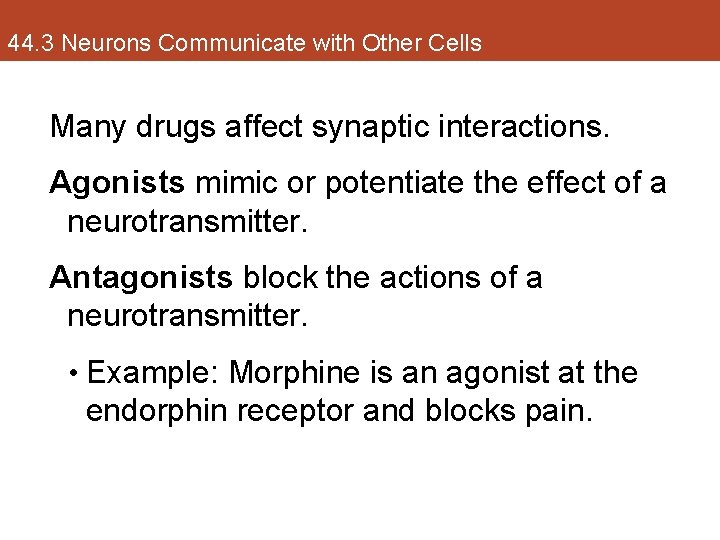
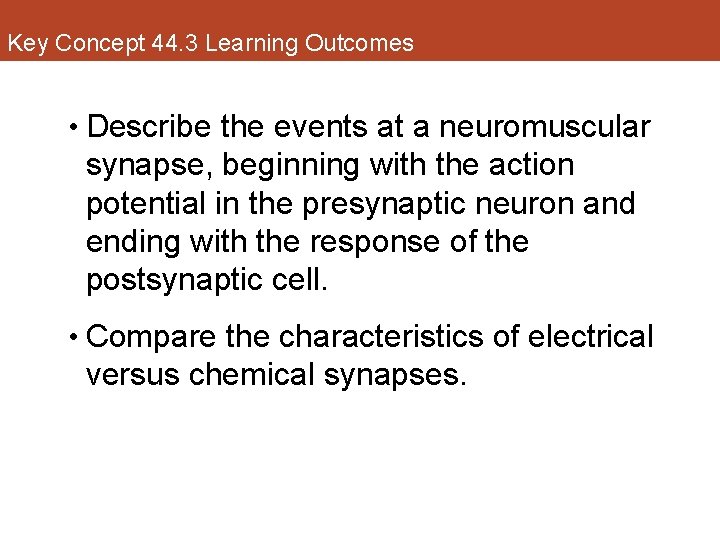
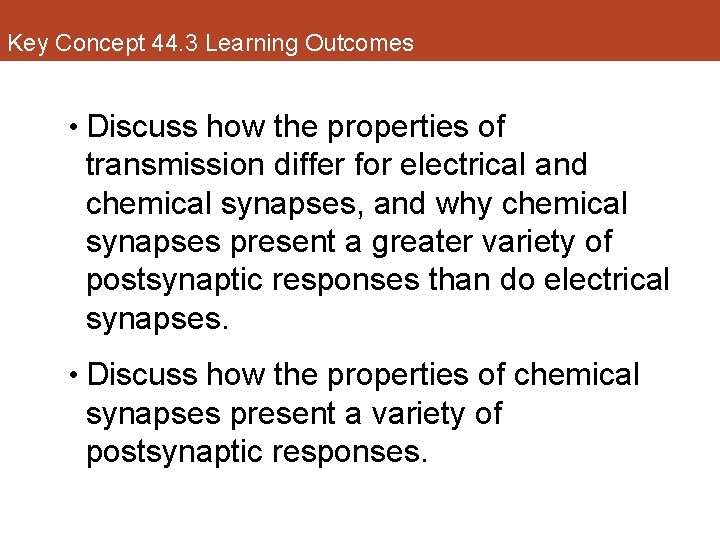
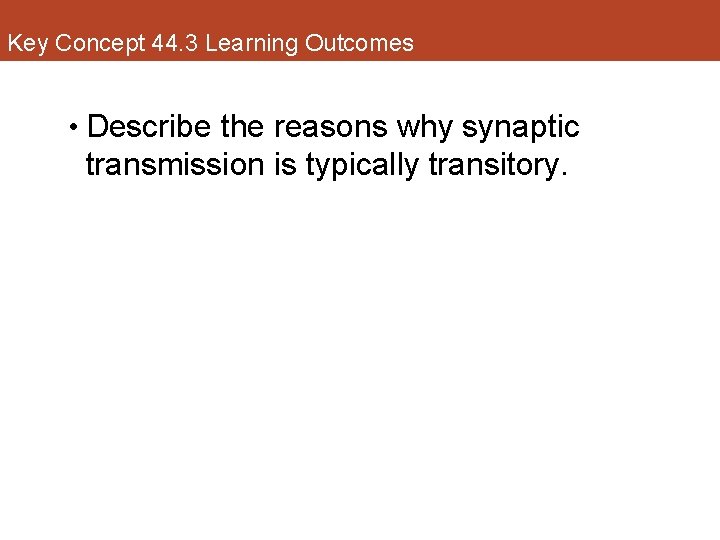
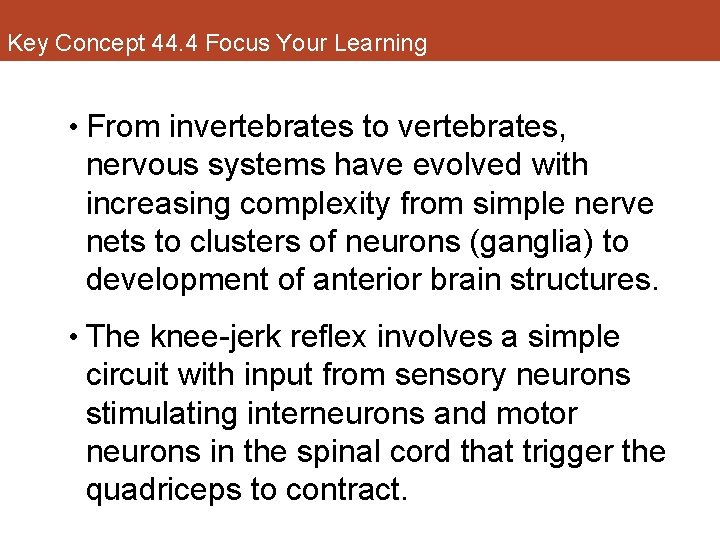
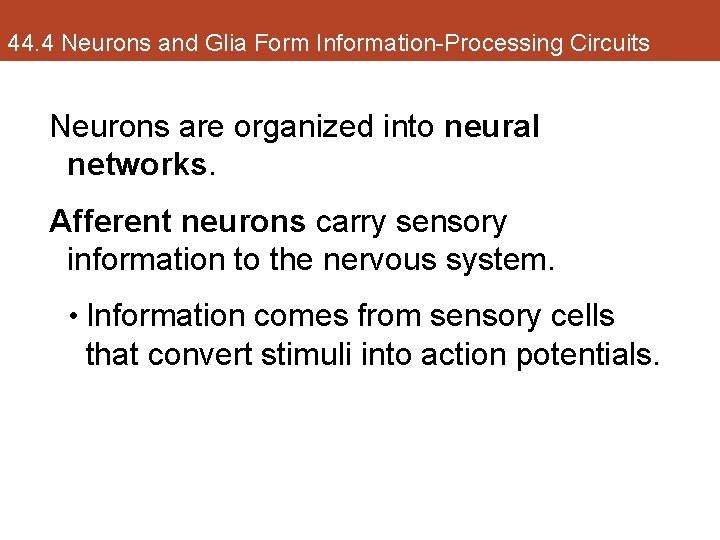
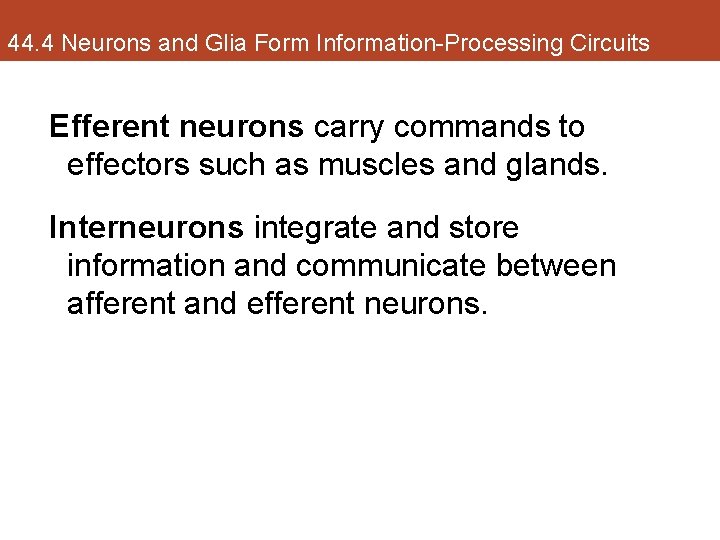
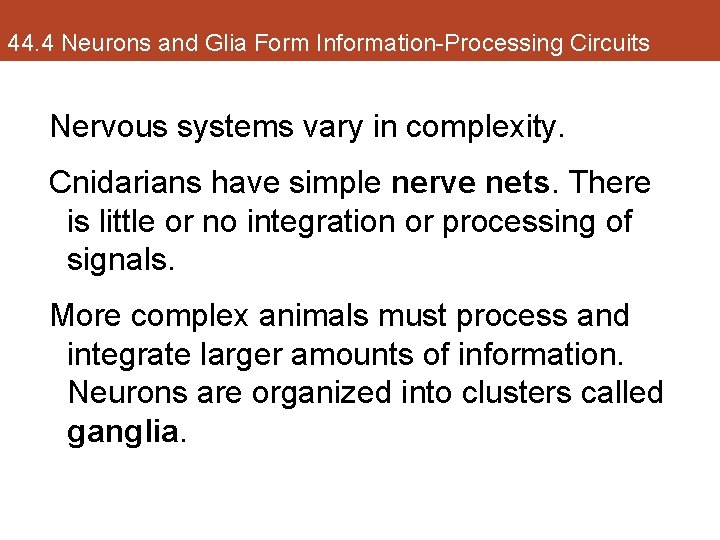
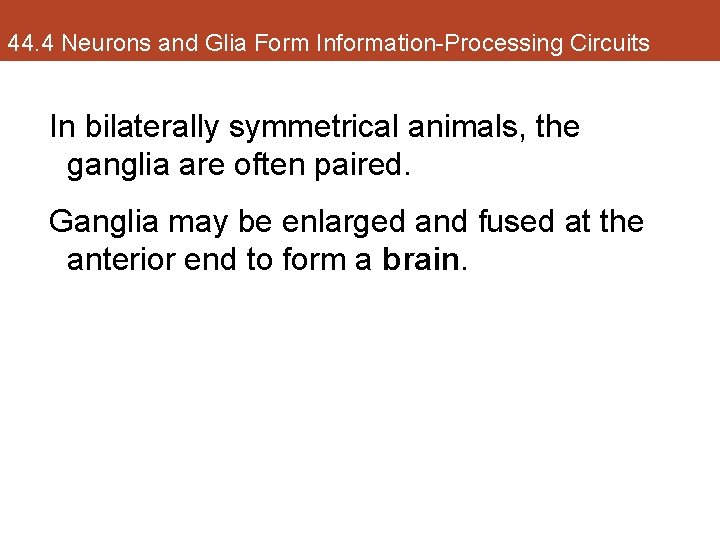
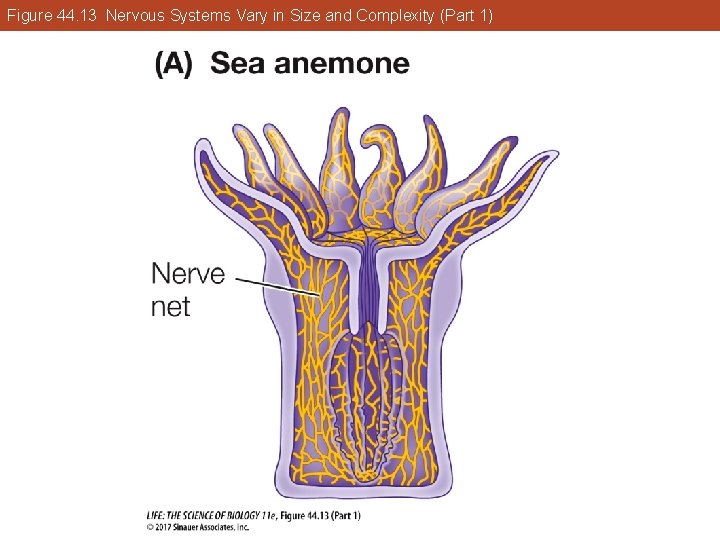
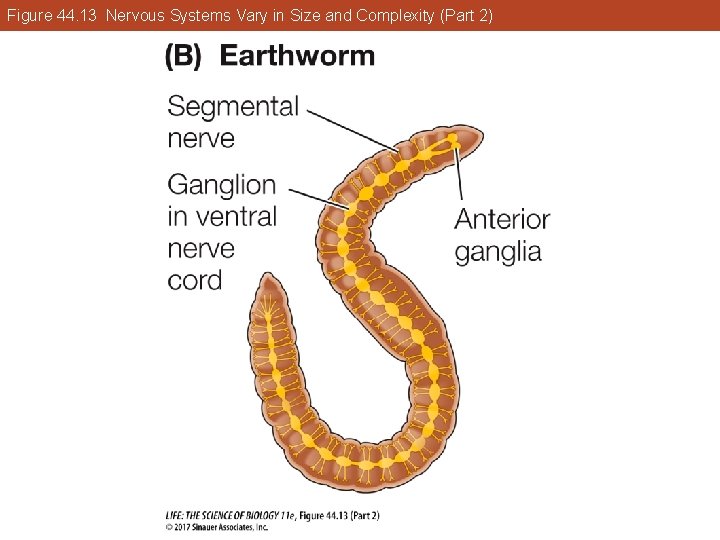
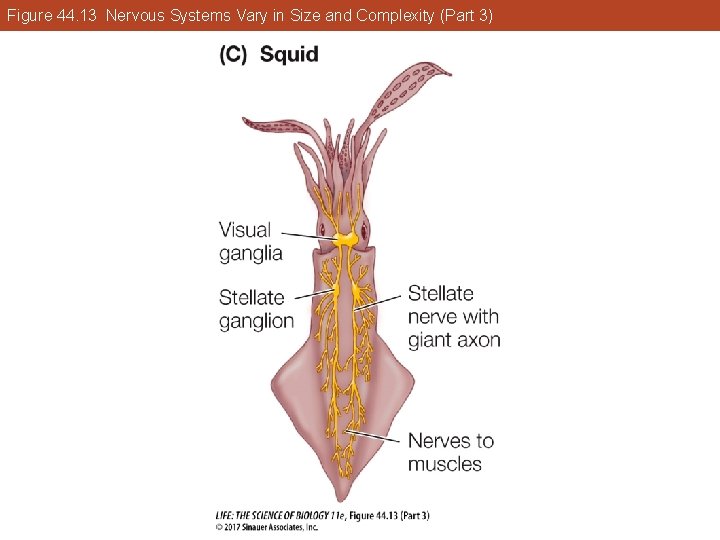
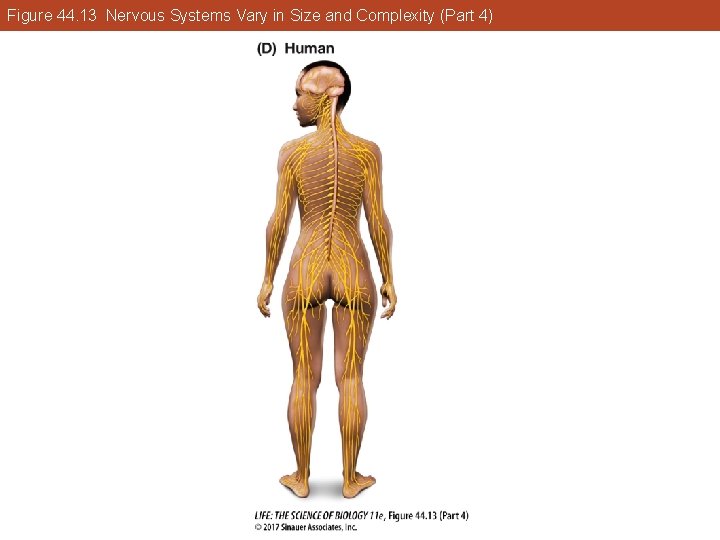
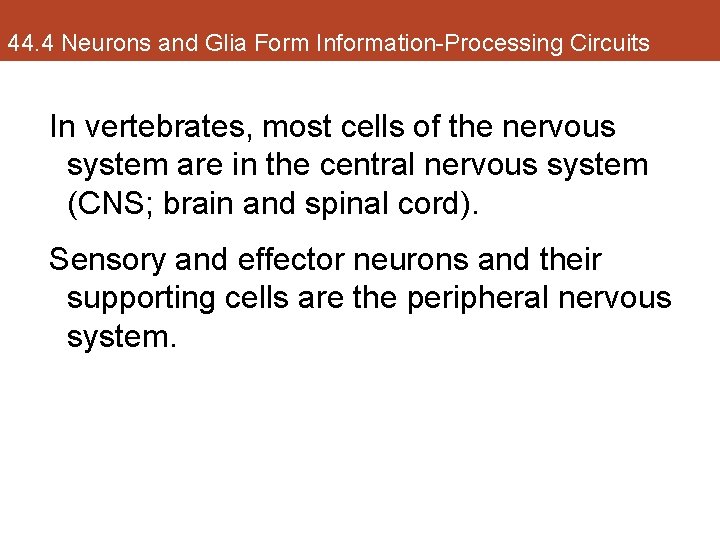
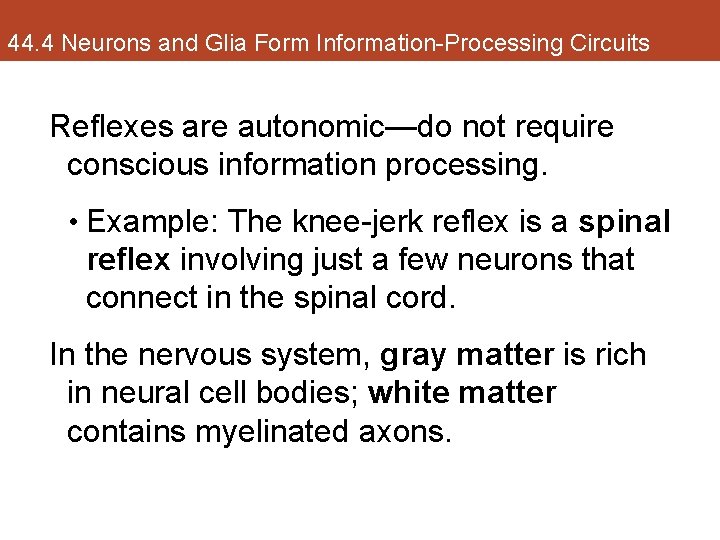
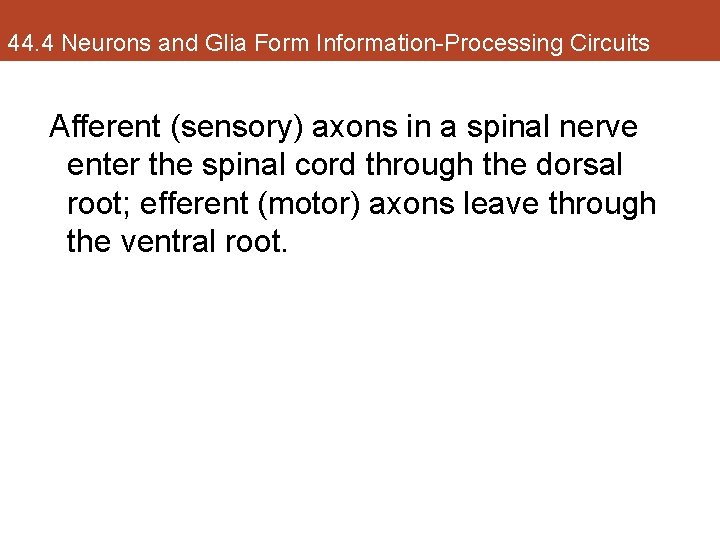
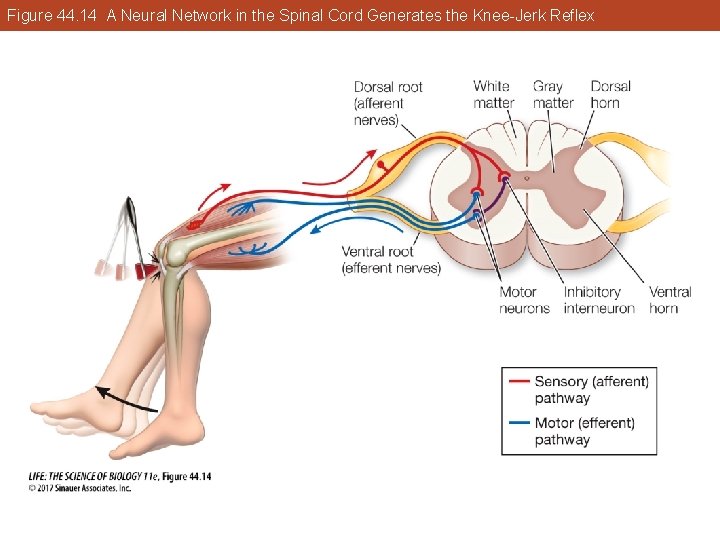
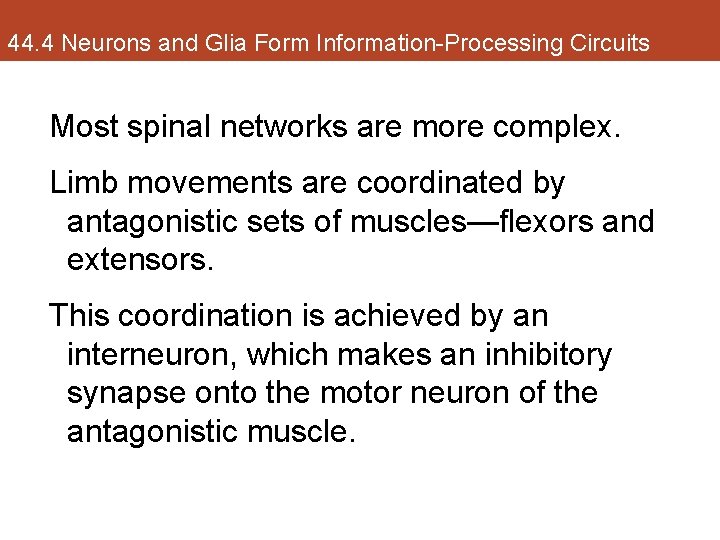
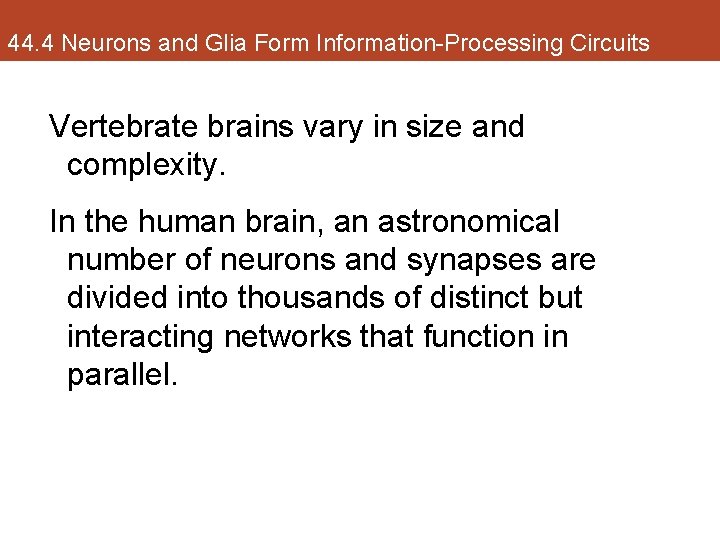
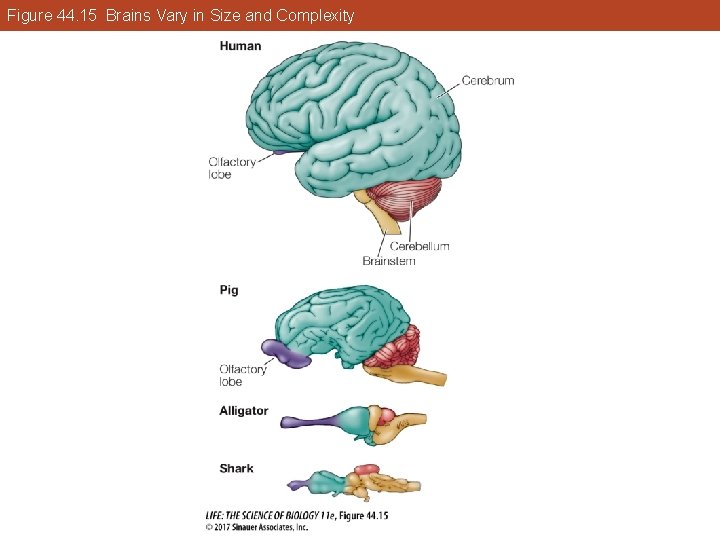
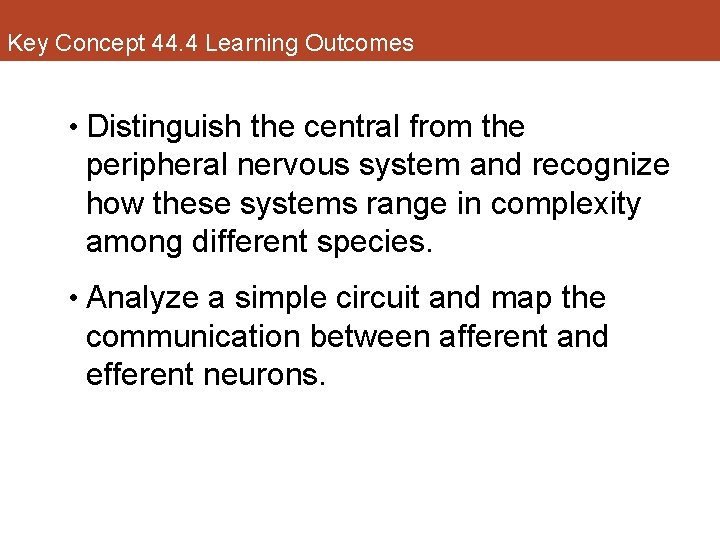
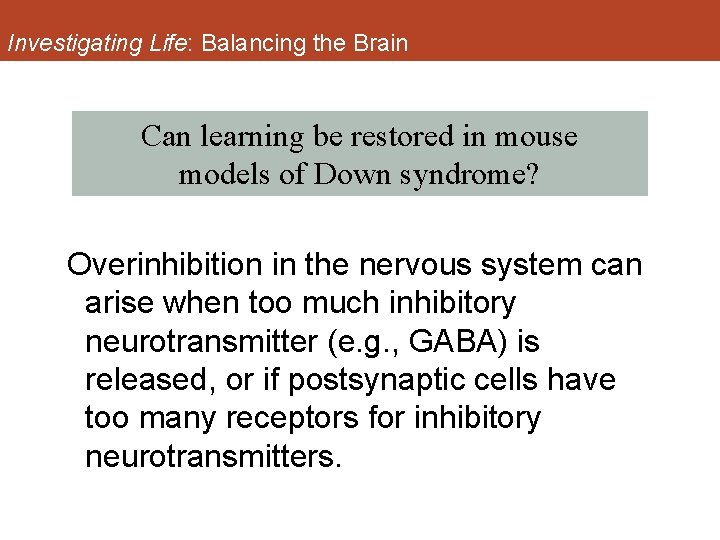
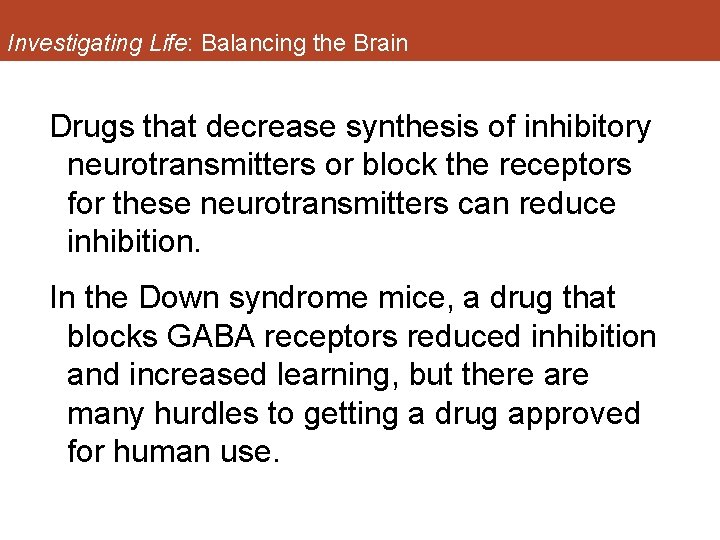
- Slides: 123
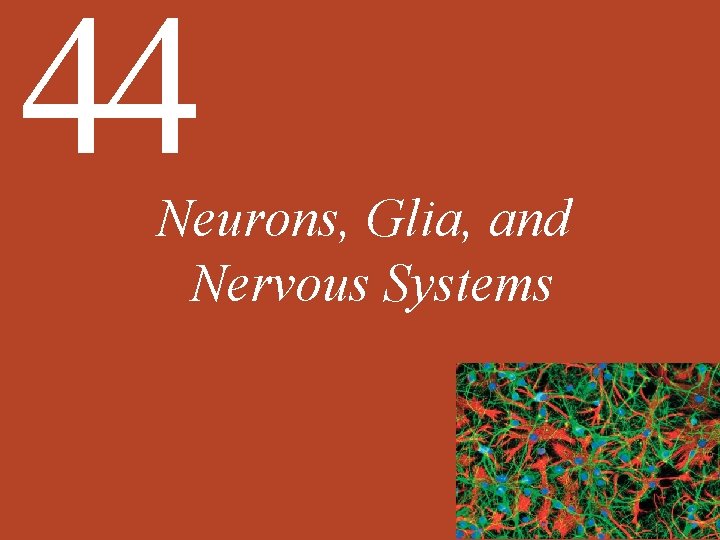
44 Neurons, Glia, and Nervous Systems
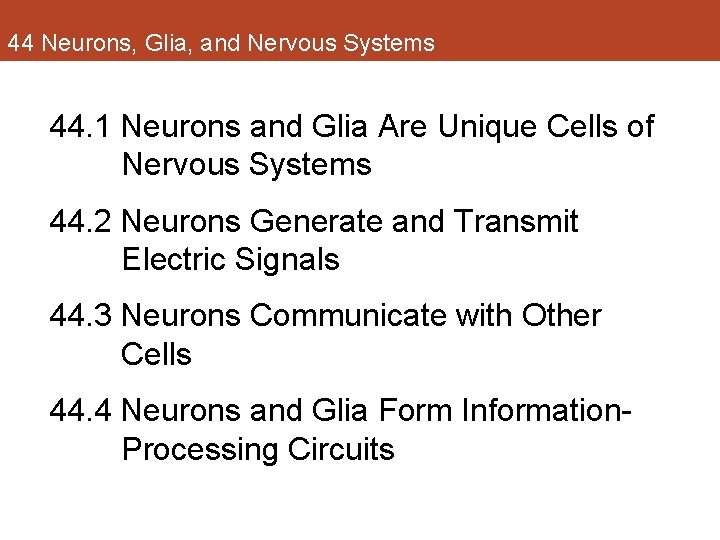
44 Neurons, Glia, and Nervous Systems 44. 1 Neurons and Glia Are Unique Cells of Nervous Systems 44. 2 Neurons Generate and Transmit Electric Signals 44. 3 Neurons Communicate with Other Cells 44. 4 Neurons and Glia Form Information. Processing Circuits
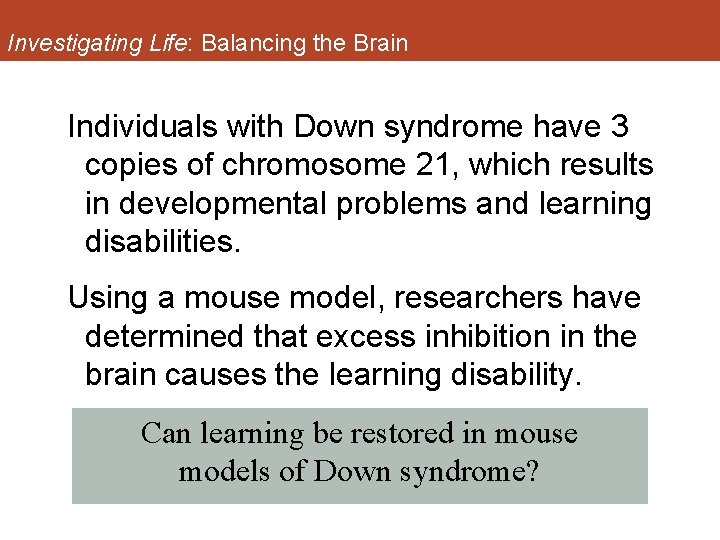
Investigating Life: Balancing the Brain Individuals with Down syndrome have 3 copies of chromosome 21, which results in developmental problems and learning disabilities. Using a mouse model, researchers have determined that excess inhibition in the brain causes the learning disability. Can learning be restored in mouse models of Down syndrome?
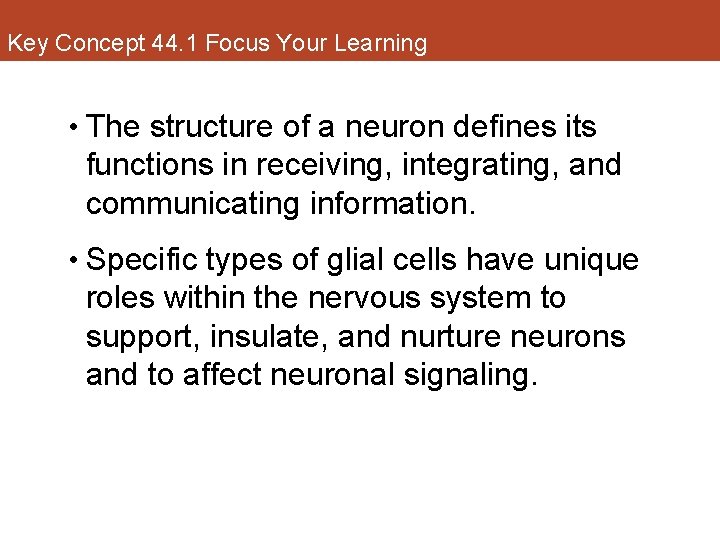
Key Concept 44. 1 Focus Your Learning • The structure of a neuron defines its functions in receiving, integrating, and communicating information. • Specific types of glial cells have unique roles within the nervous system to support, insulate, and nurture neurons and to affect neuronal signaling.
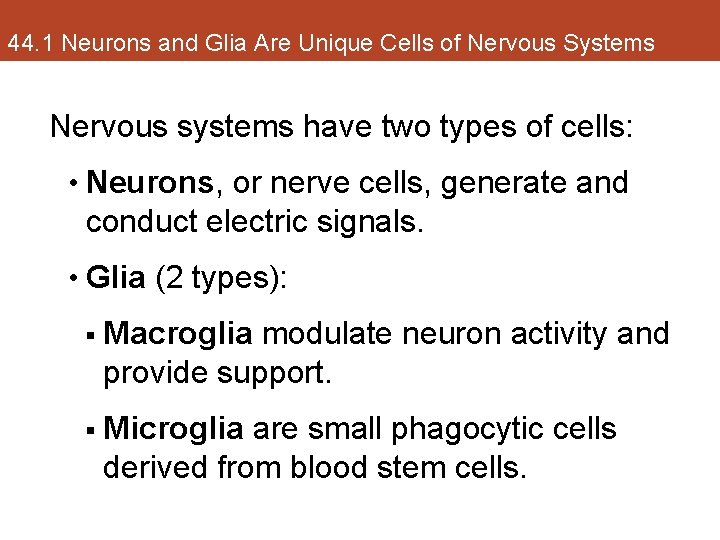
44. 1 Neurons and Glia Are Unique Cells of Nervous Systems Nervous systems have two types of cells: • Neurons, or nerve cells, generate and conduct electric signals. • Glia (2 types): § Macroglia modulate neuron activity and provide support. § Microglia are small phagocytic cells derived from blood stem cells.
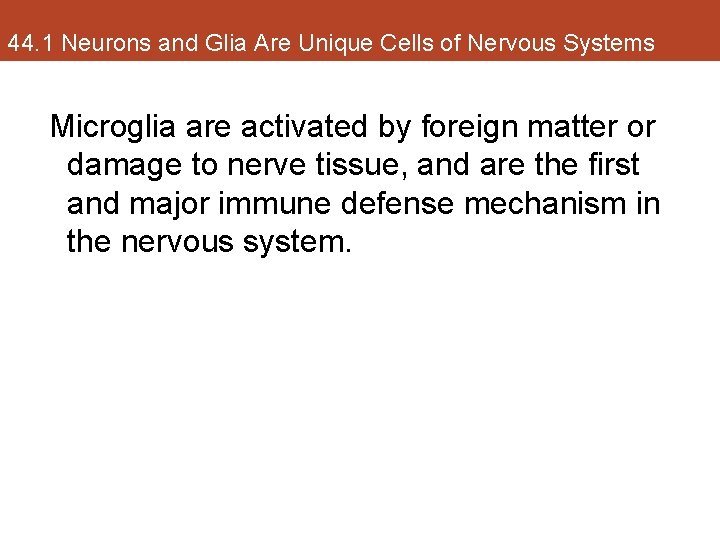
44. 1 Neurons and Glia Are Unique Cells of Nervous Systems Microglia are activated by foreign matter or damage to nerve tissue, and are the first and major immune defense mechanism in the nervous system.
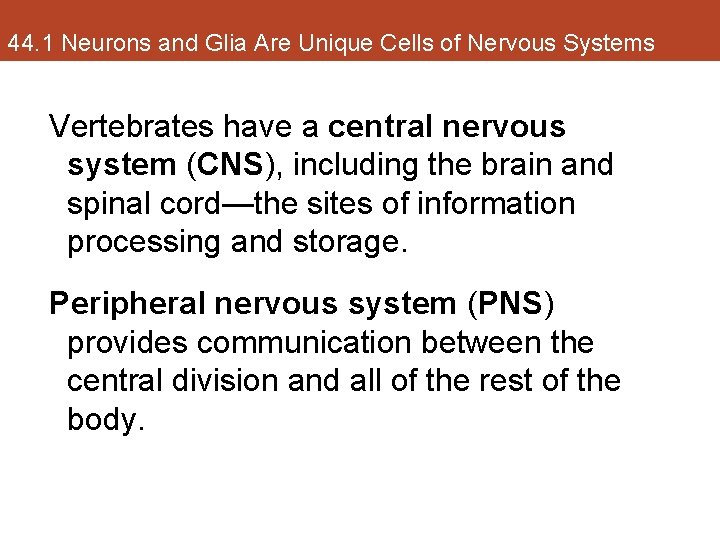
44. 1 Neurons and Glia Are Unique Cells of Nervous Systems Vertebrates have a central nervous system (CNS), including the brain and spinal cord—the sites of information processing and storage. Peripheral nervous system (PNS) provides communication between the central division and all of the rest of the body.
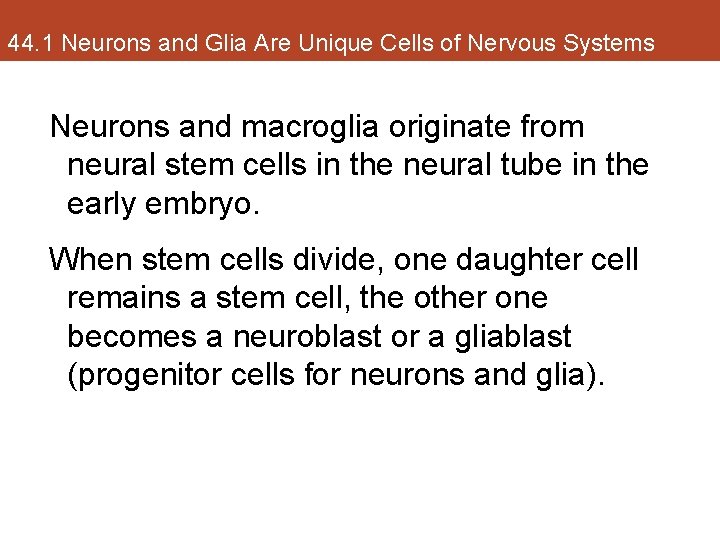
44. 1 Neurons and Glia Are Unique Cells of Nervous Systems Neurons and macroglia originate from neural stem cells in the neural tube in the early embryo. When stem cells divide, one daughter cell remains a stem cell, the other one becomes a neuroblast or a gliablast (progenitor cells for neurons and glia).
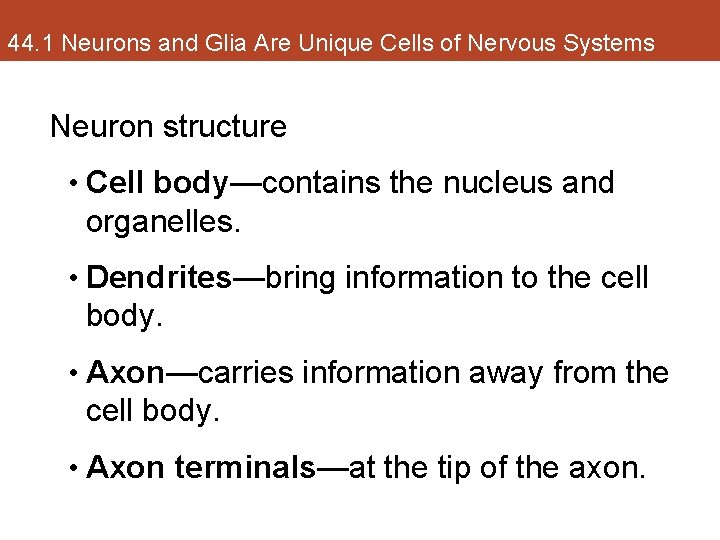
44. 1 Neurons and Glia Are Unique Cells of Nervous Systems Neuron structure • Cell body—contains the nucleus and organelles. • Dendrites—bring information to the cell body. • Axon—carries information away from the cell body. • Axon terminals—at the tip of the axon.
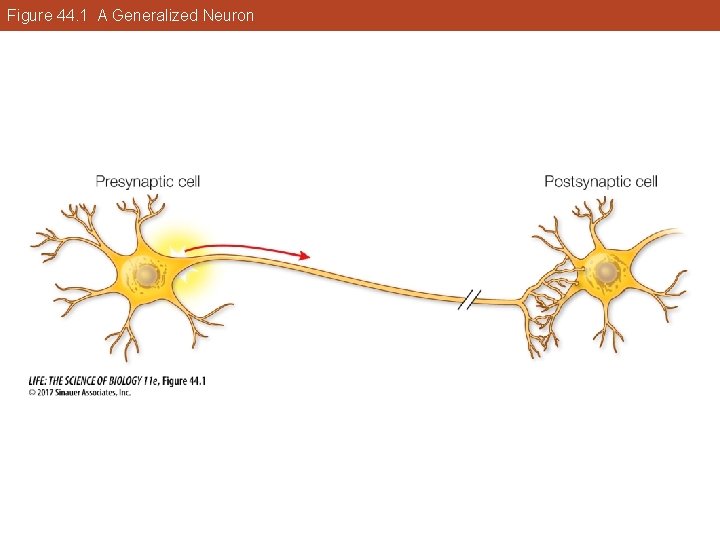
Figure 44. 1 A Generalized Neuron
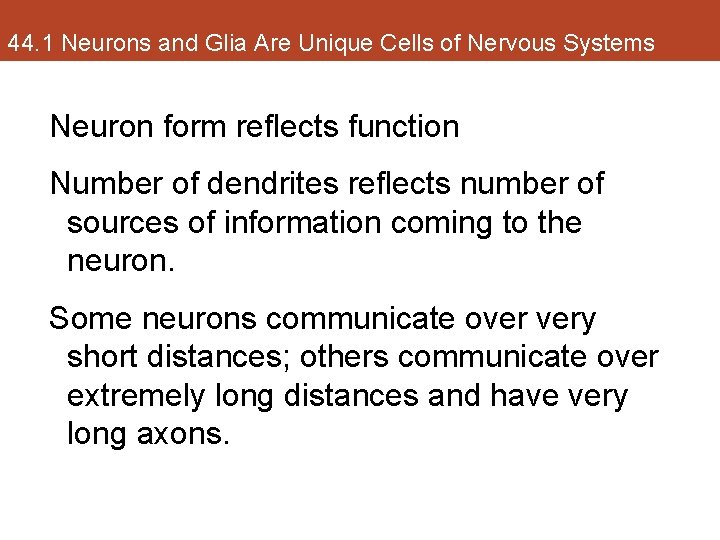
44. 1 Neurons and Glia Are Unique Cells of Nervous Systems Neuron form reflects function Number of dendrites reflects number of sources of information coming to the neuron. Some neurons communicate over very short distances; others communicate over extremely long distances and have very long axons.
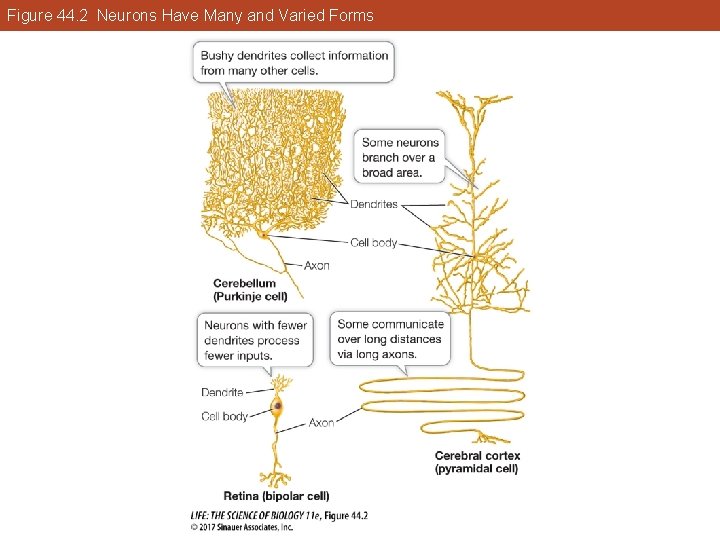
Figure 44. 2 Neurons Have Many and Varied Forms
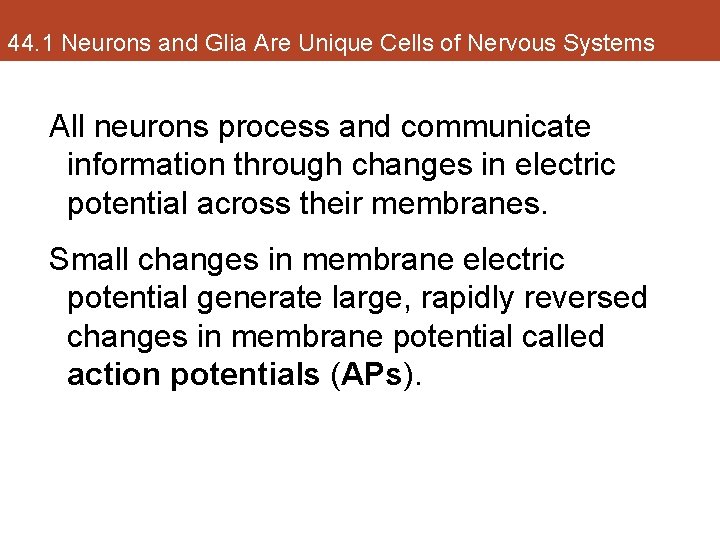
44. 1 Neurons and Glia Are Unique Cells of Nervous Systems All neurons process and communicate information through changes in electric potential across their membranes. Small changes in membrane electric potential generate large, rapidly reversed changes in membrane potential called action potentials (APs).
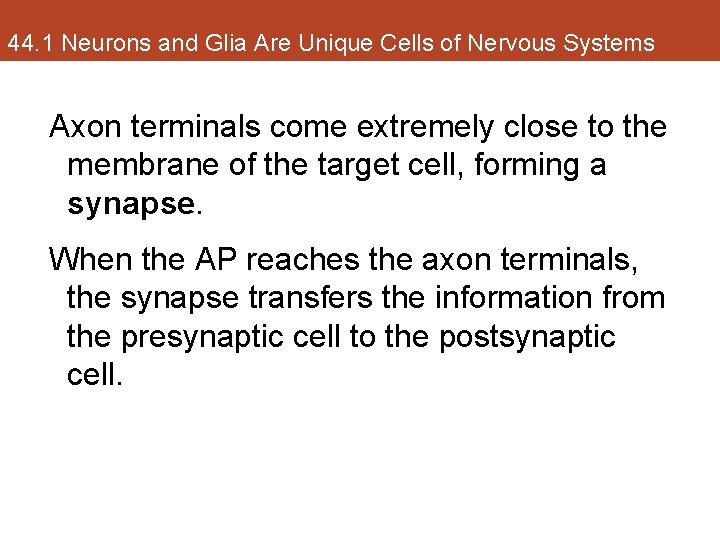
44. 1 Neurons and Glia Are Unique Cells of Nervous Systems Axon terminals come extremely close to the membrane of the target cell, forming a synapse. When the AP reaches the axon terminals, the synapse transfers the information from the presynaptic cell to the postsynaptic cell.
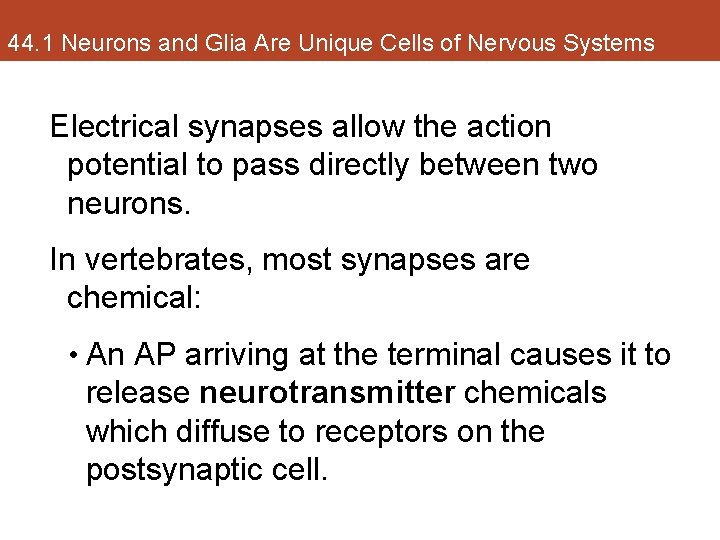
44. 1 Neurons and Glia Are Unique Cells of Nervous Systems Electrical synapses allow the action potential to pass directly between two neurons. In vertebrates, most synapses are chemical: • An AP arriving at the terminal causes it to release neurotransmitter chemicals which diffuse to receptors on the postsynaptic cell.
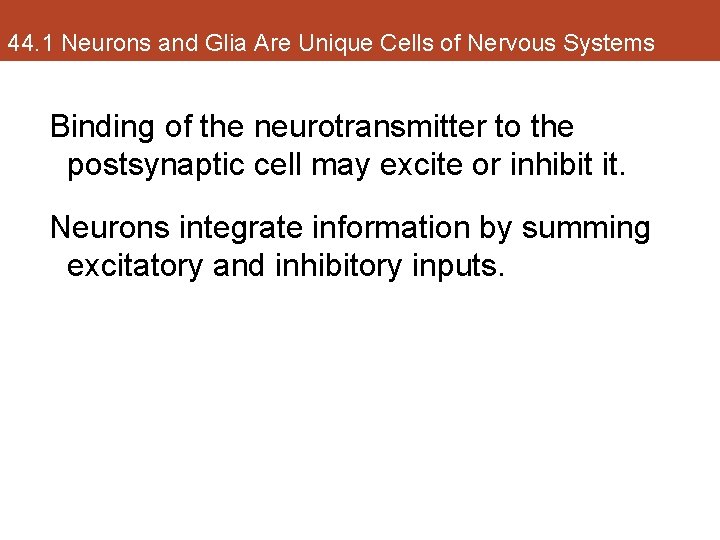
44. 1 Neurons and Glia Are Unique Cells of Nervous Systems Binding of the neurotransmitter to the postsynaptic cell may excite or inhibit it. Neurons integrate information by summing excitatory and inhibitory inputs.
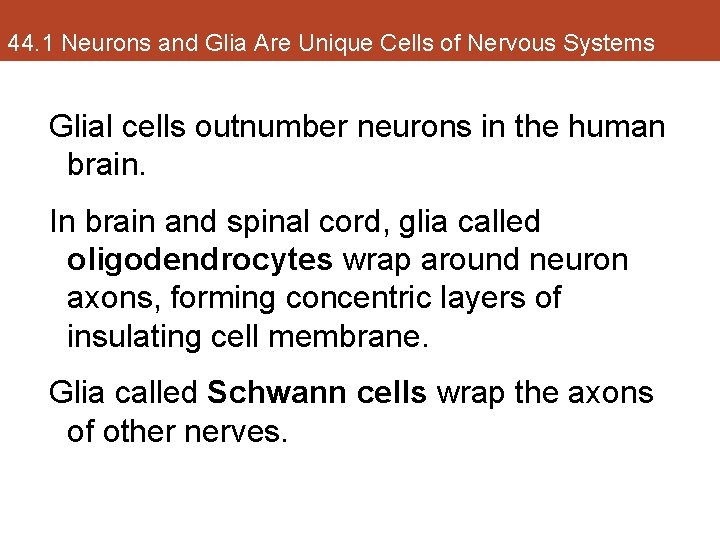
44. 1 Neurons and Glia Are Unique Cells of Nervous Systems Glial cells outnumber neurons in the human brain. In brain and spinal cord, glia called oligodendrocytes wrap around neuron axons, forming concentric layers of insulating cell membrane. Glia called Schwann cells wrap the axons of other nerves.
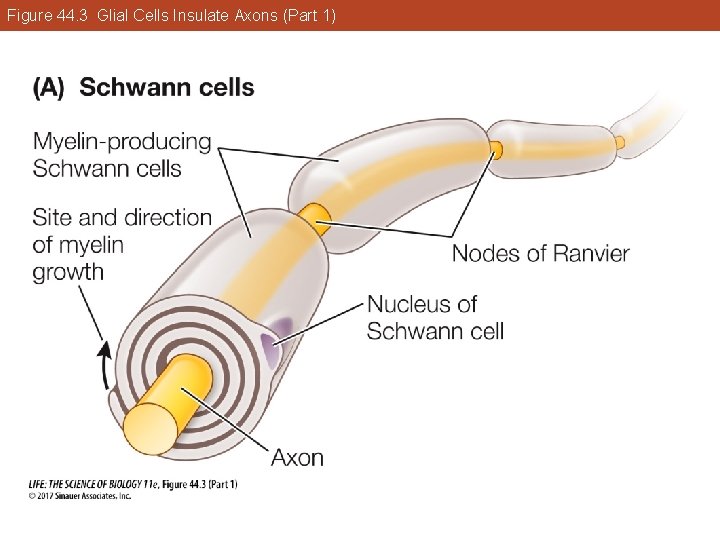
Figure 44. 3 Glial Cells Insulate Axons (Part 1)
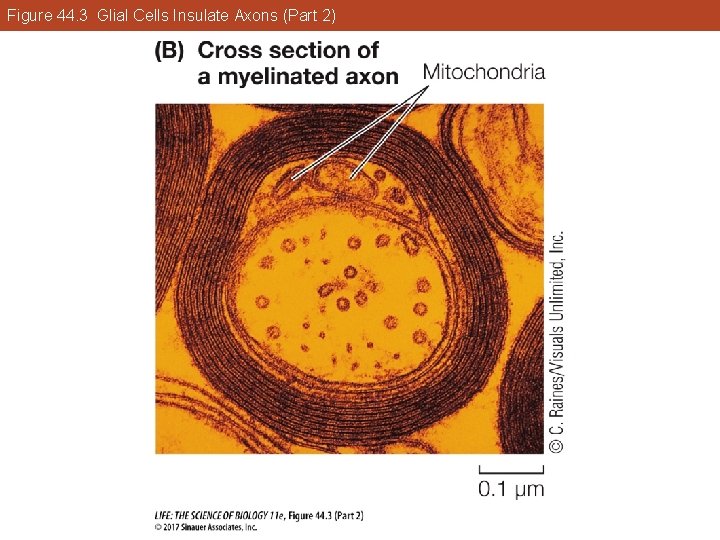
Figure 44. 3 Glial Cells Insulate Axons (Part 2)
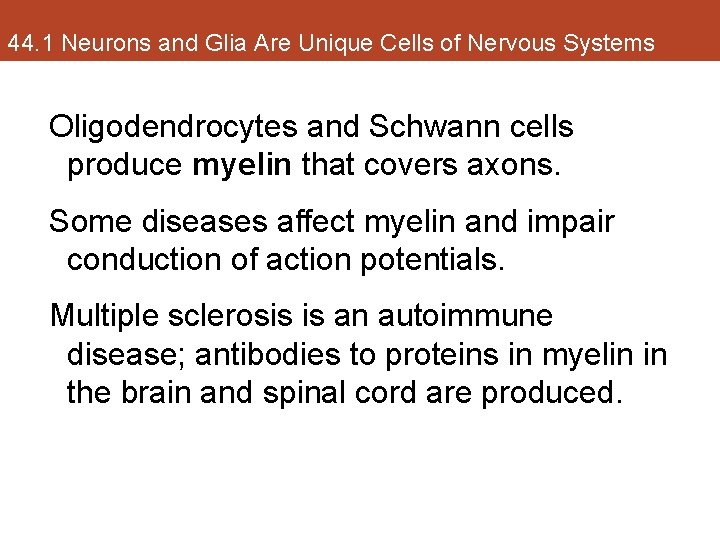
44. 1 Neurons and Glia Are Unique Cells of Nervous Systems Oligodendrocytes and Schwann cells produce myelin that covers axons. Some diseases affect myelin and impair conduction of action potentials. Multiple sclerosis is an autoimmune disease; antibodies to proteins in myelin in the brain and spinal cord are produced.
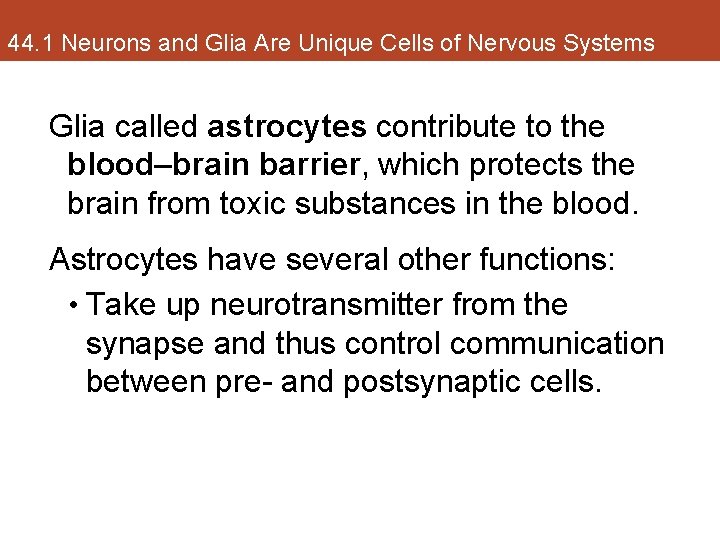
44. 1 Neurons and Glia Are Unique Cells of Nervous Systems Glia called astrocytes contribute to the blood–brain barrier, which protects the brain from toxic substances in the blood. Astrocytes have several other functions: • Take up neurotransmitter from the synapse and thus control communication between pre- and postsynaptic cells.
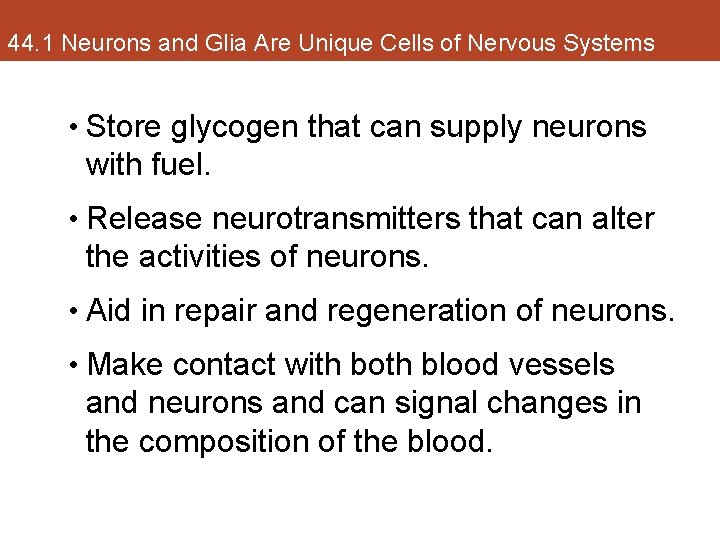
44. 1 Neurons and Glia Are Unique Cells of Nervous Systems • Store glycogen that can supply neurons with fuel. • Release neurotransmitters that can alter the activities of neurons. • Aid in repair and regeneration of neurons. • Make contact with both blood vessels and neurons and can signal changes in the composition of the blood.
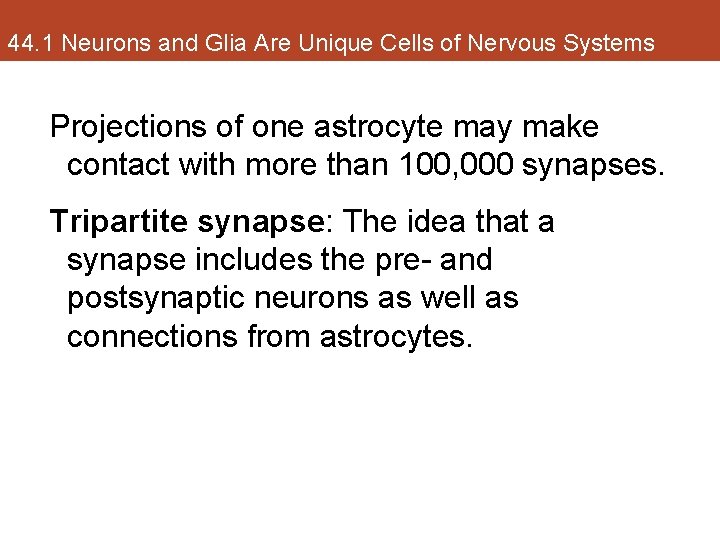
44. 1 Neurons and Glia Are Unique Cells of Nervous Systems Projections of one astrocyte may make contact with more than 100, 000 synapses. Tripartite synapse: The idea that a synapse includes the pre- and postsynaptic neurons as well as connections from astrocytes.
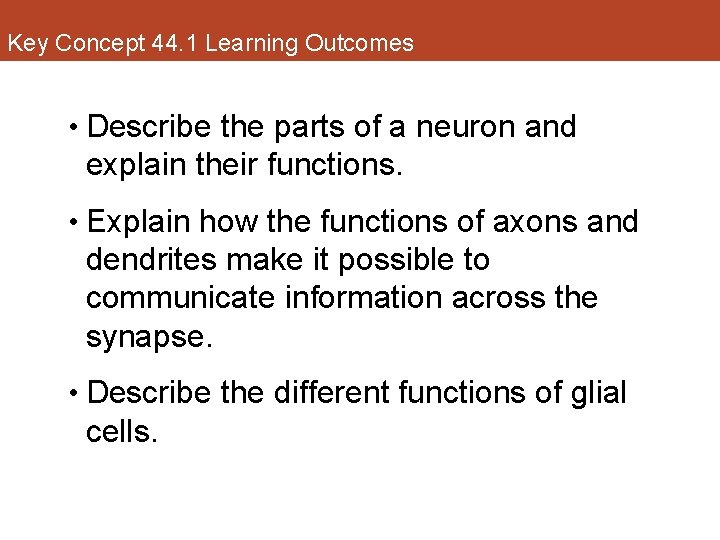
Key Concept 44. 1 Learning Outcomes • Describe the parts of a neuron and explain their functions. • Explain how the functions of axons and dendrites make it possible to communicate information across the synapse. • Describe the different functions of glial cells.
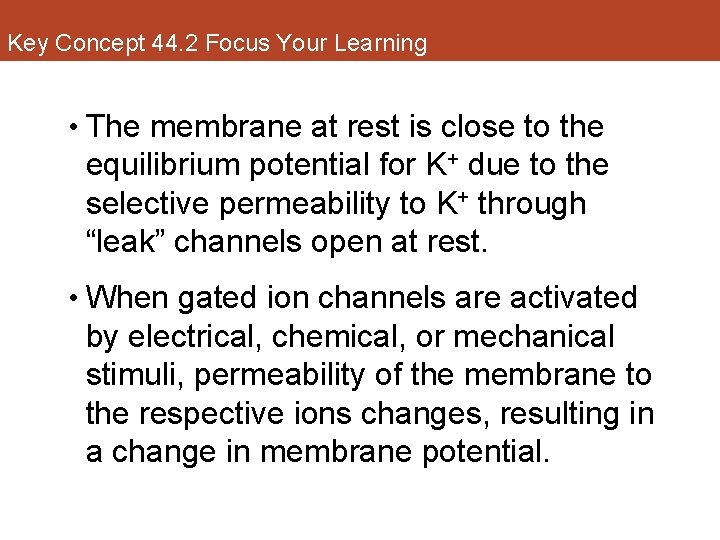
Key Concept 44. 2 Focus Your Learning • The membrane at rest is close to the equilibrium potential for K+ due to the selective permeability to K+ through “leak” channels open at rest. • When gated ion channels are activated by electrical, chemical, or mechanical stimuli, permeability of the membrane to the respective ions changes, resulting in a change in membrane potential.
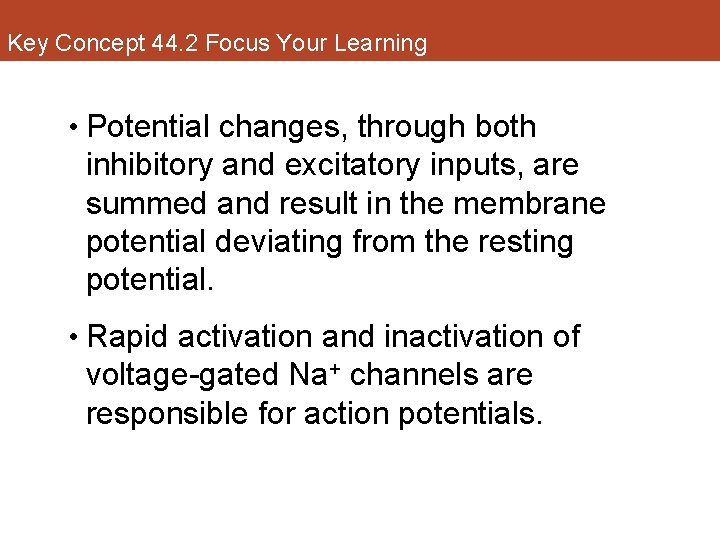
Key Concept 44. 2 Focus Your Learning • Potential changes, through both inhibitory and excitatory inputs, are summed and result in the membrane potential deviating from the resting potential. • Rapid activation and inactivation of voltage-gated Na+ channels are responsible for action potentials.
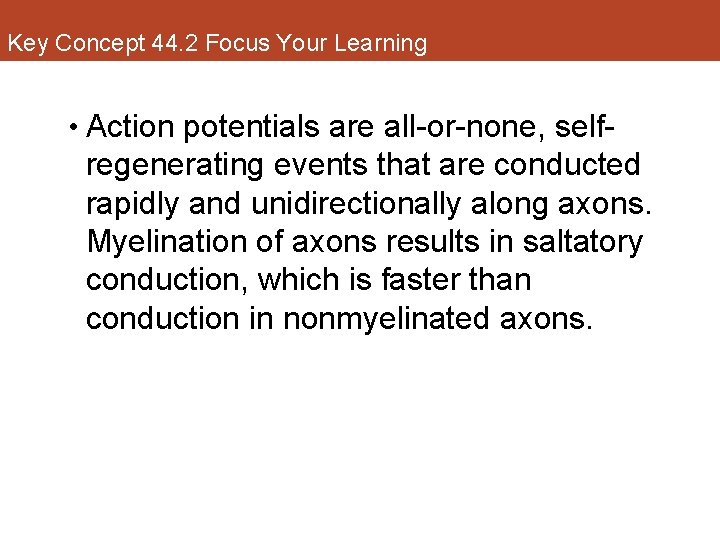
Key Concept 44. 2 Focus Your Learning • Action potentials are all-or-none, self- regenerating events that are conducted rapidly and unidirectionally along axons. Myelination of axons results in saltatory conduction, which is faster than conduction in nonmyelinated axons.
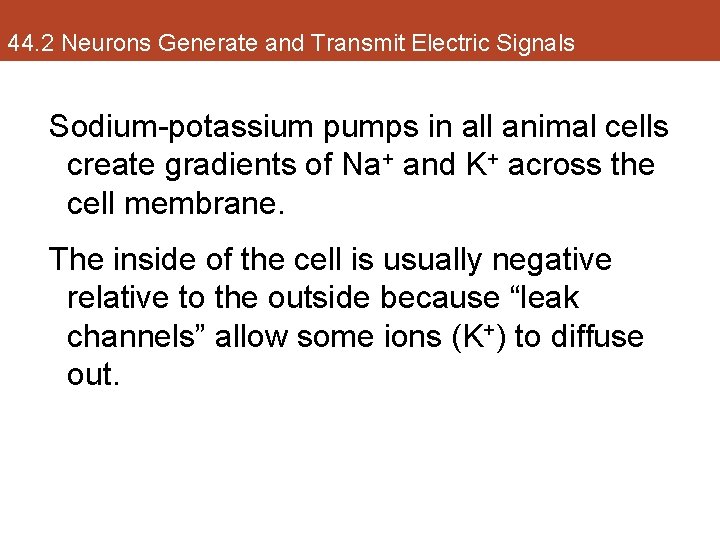
44. 2 Neurons Generate and Transmit Electric Signals Sodium-potassium pumps in all animal cells create gradients of Na+ and K+ across the cell membrane. The inside of the cell is usually negative relative to the outside because “leak channels” allow some ions (K+) to diffuse out.
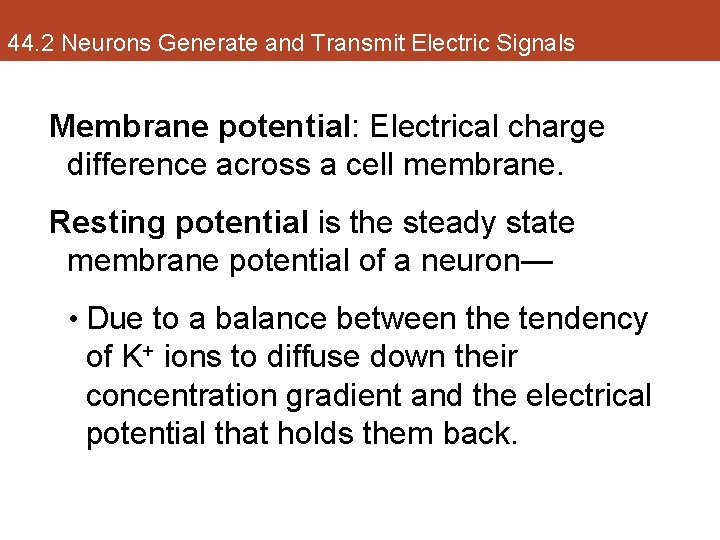
44. 2 Neurons Generate and Transmit Electric Signals Membrane potential: Electrical charge difference across a cell membrane. Resting potential is the steady state membrane potential of a neuron— • Due to a balance between the tendency of K+ ions to diffuse down their concentration gradient and the electrical potential that holds them back.
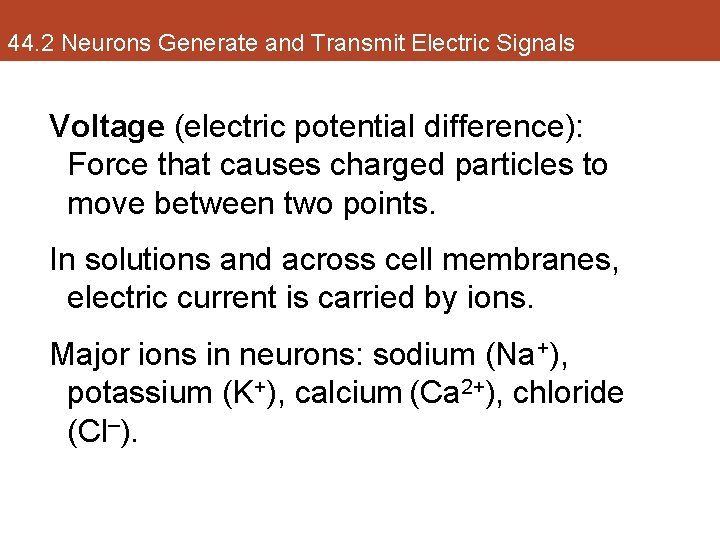
44. 2 Neurons Generate and Transmit Electric Signals Voltage (electric potential difference): Force that causes charged particles to move between two points. In solutions and across cell membranes, electric current is carried by ions. Major ions in neurons: sodium (Na+), potassium (K+), calcium (Ca 2+), chloride (Cl–).
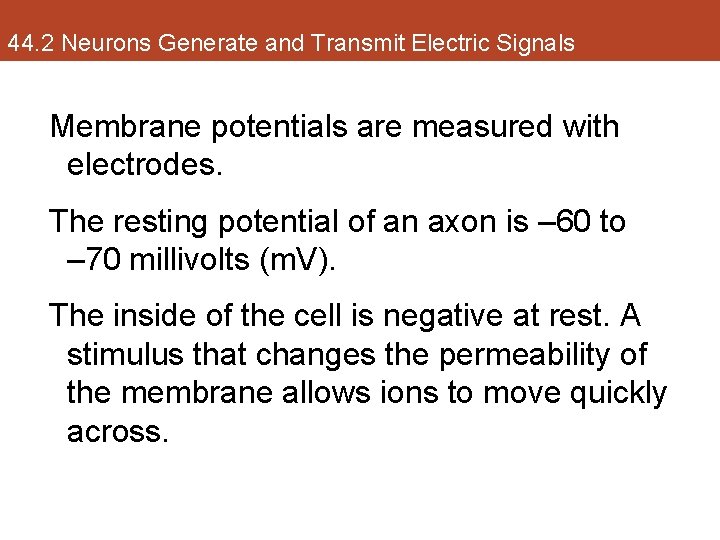
44. 2 Neurons Generate and Transmit Electric Signals Membrane potentials are measured with electrodes. The resting potential of an axon is – 60 to – 70 millivolts (m. V). The inside of the cell is negative at rest. A stimulus that changes the permeability of the membrane allows ions to move quickly across.
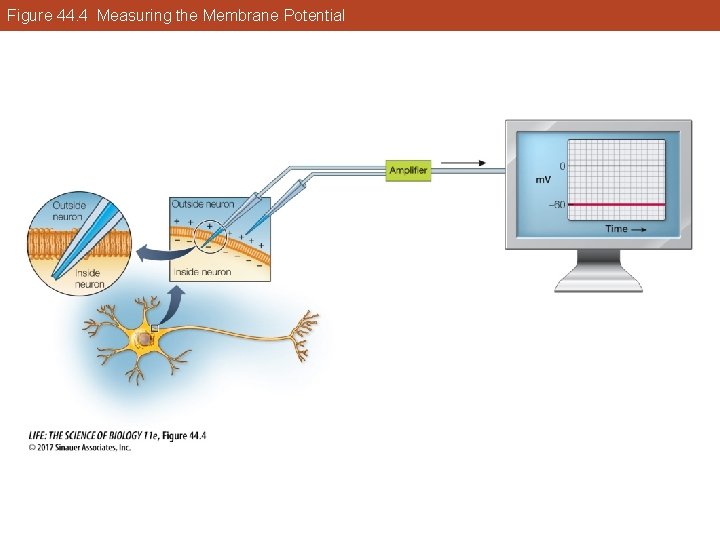
Figure 44. 4 Measuring the Membrane Potential
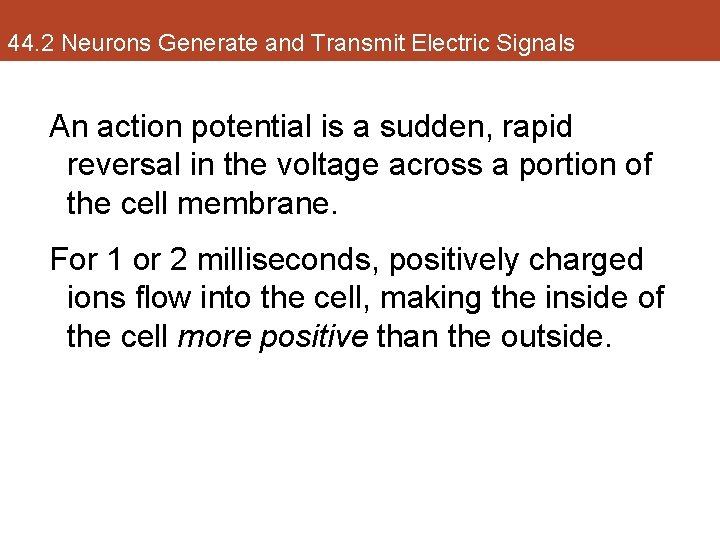
44. 2 Neurons Generate and Transmit Electric Signals An action potential is a sudden, rapid reversal in the voltage across a portion of the cell membrane. For 1 or 2 milliseconds, positively charged ions flow into the cell, making the inside of the cell more positive than the outside.
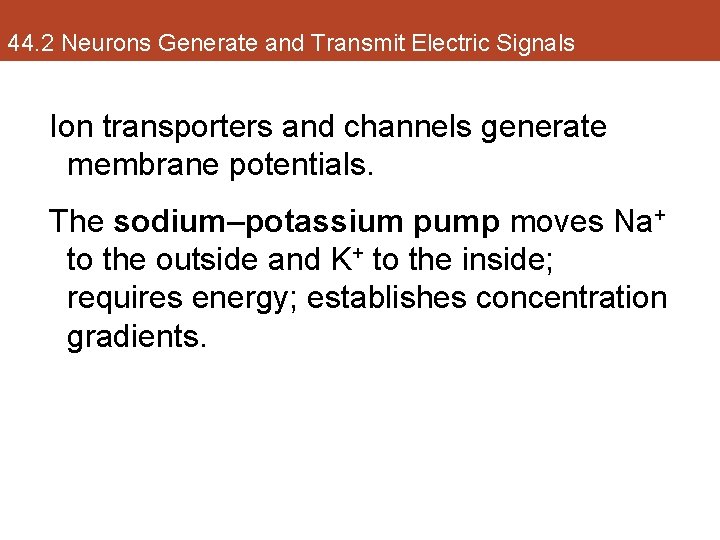
44. 2 Neurons Generate and Transmit Electric Signals Ion transporters and channels generate membrane potentials. The sodium–potassium pump moves Na+ to the outside and K+ to the inside; requires energy; establishes concentration gradients.
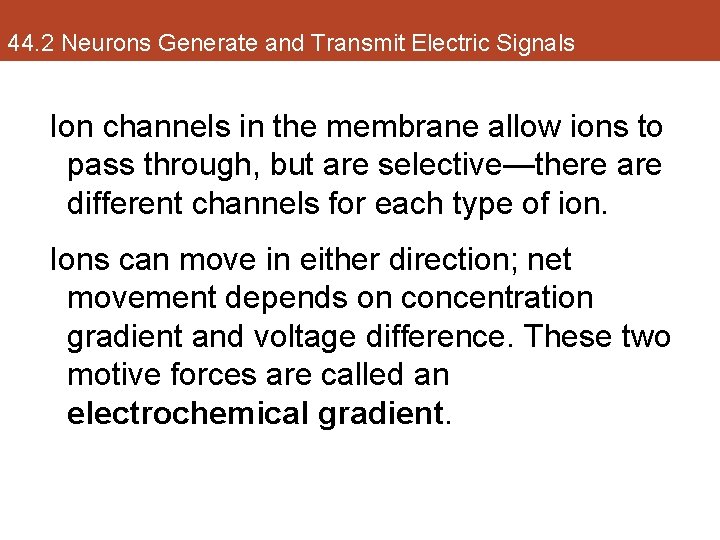
44. 2 Neurons Generate and Transmit Electric Signals Ion channels in the membrane allow ions to pass through, but are selective—there are different channels for each type of ion. Ions can move in either direction; net movement depends on concentration gradient and voltage difference. These two motive forces are called an electrochemical gradient.
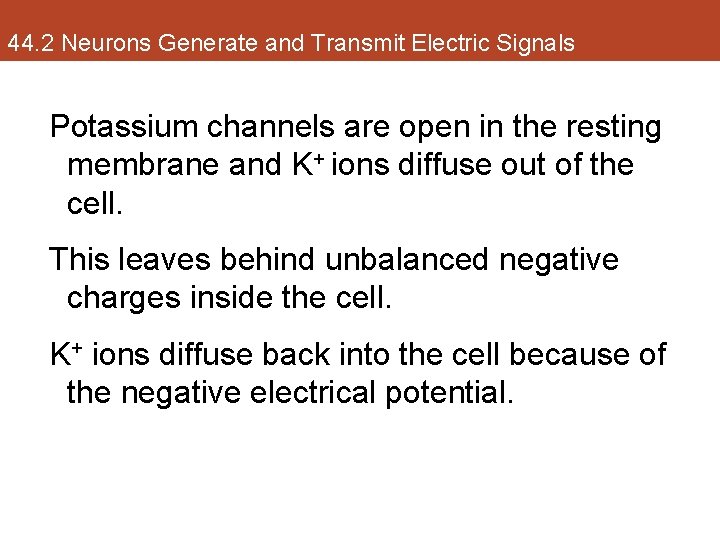
44. 2 Neurons Generate and Transmit Electric Signals Potassium channels are open in the resting membrane and K+ ions diffuse out of the cell. This leaves behind unbalanced negative charges inside the cell. K+ ions diffuse back into the cell because of the negative electrical potential.
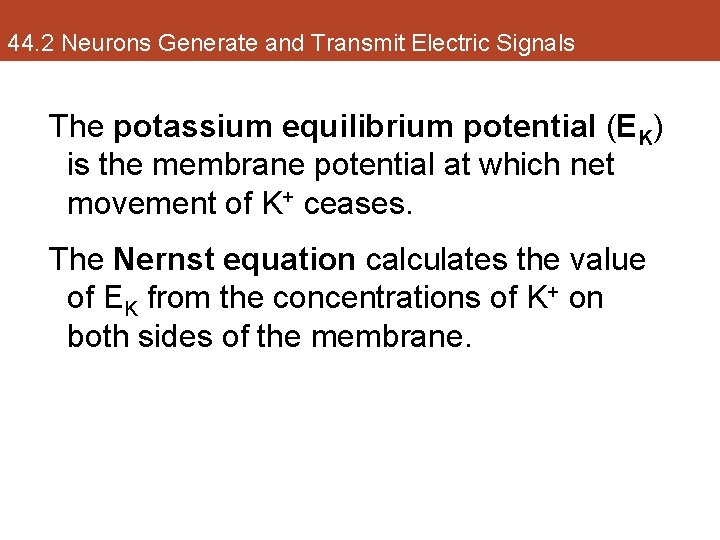
44. 2 Neurons Generate and Transmit Electric Signals The potassium equilibrium potential (EK) is the membrane potential at which net movement of K+ ceases. The Nernst equation calculates the value of EK from the concentrations of K+ on both sides of the membrane.
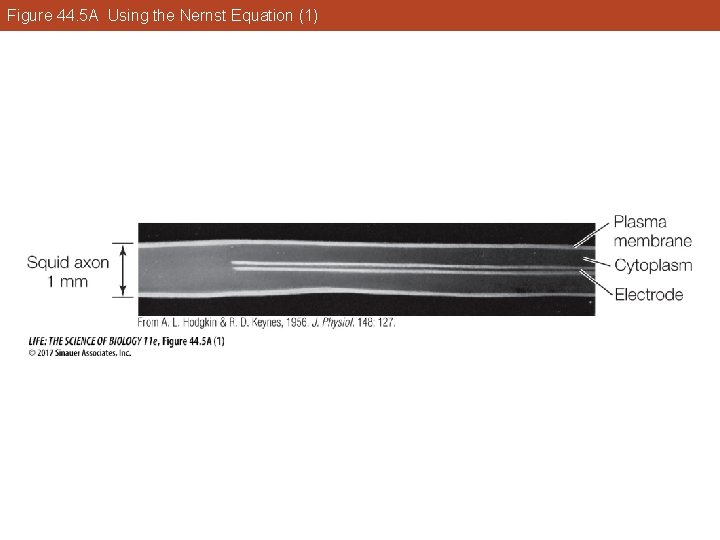
Figure 44. 5 A Using the Nernst Equation (1)
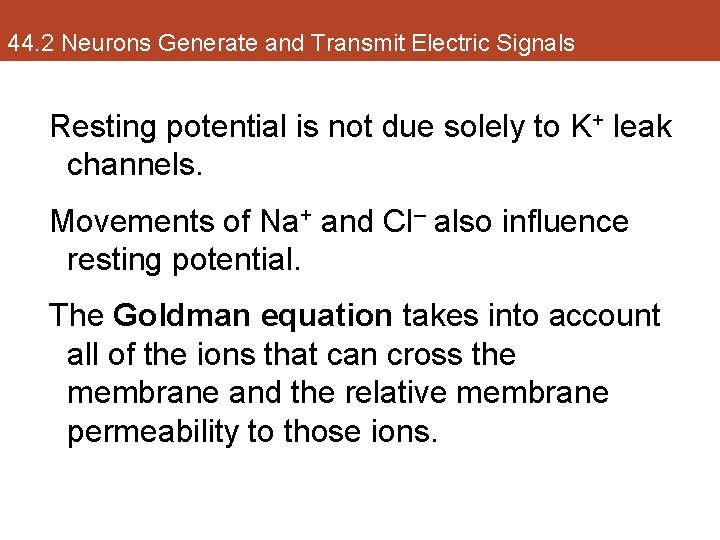
44. 2 Neurons Generate and Transmit Electric Signals Resting potential is not due solely to K+ leak channels. Movements of Na+ and Cl– also influence resting potential. The Goldman equation takes into account all of the ions that can cross the membrane and the relative membrane permeability to those ions.
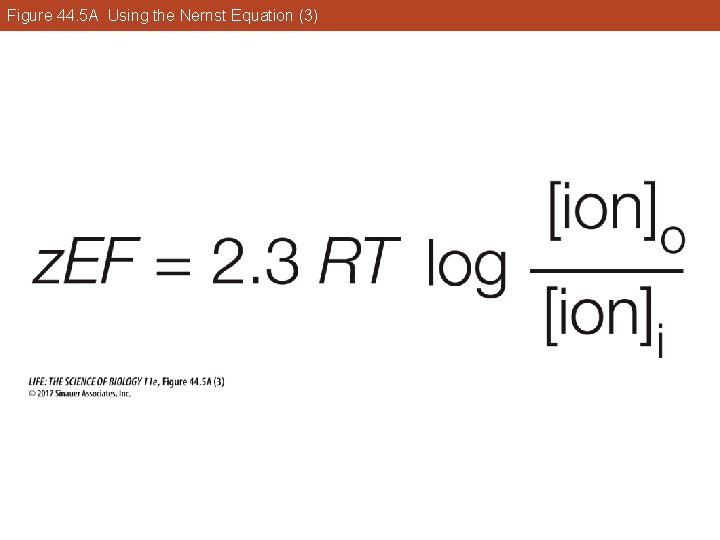
Figure 44. 5 A Using the Nernst Equation (3)
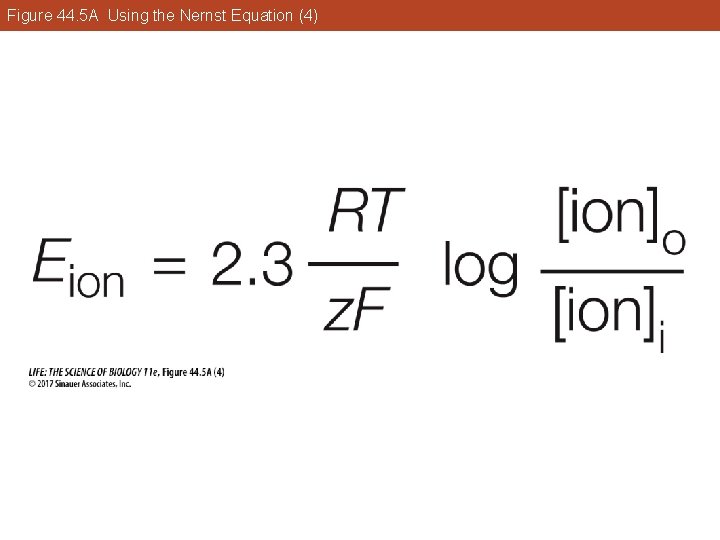
Figure 44. 5 A Using the Nernst Equation (4)
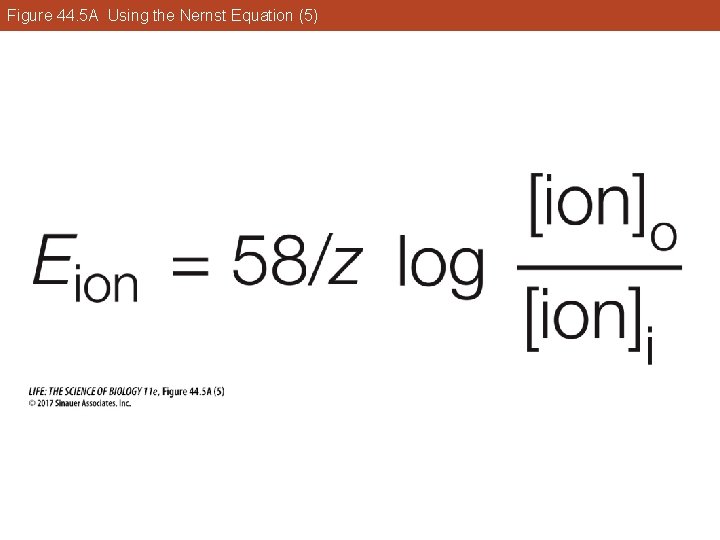
Figure 44. 5 A Using the Nernst Equation (5)
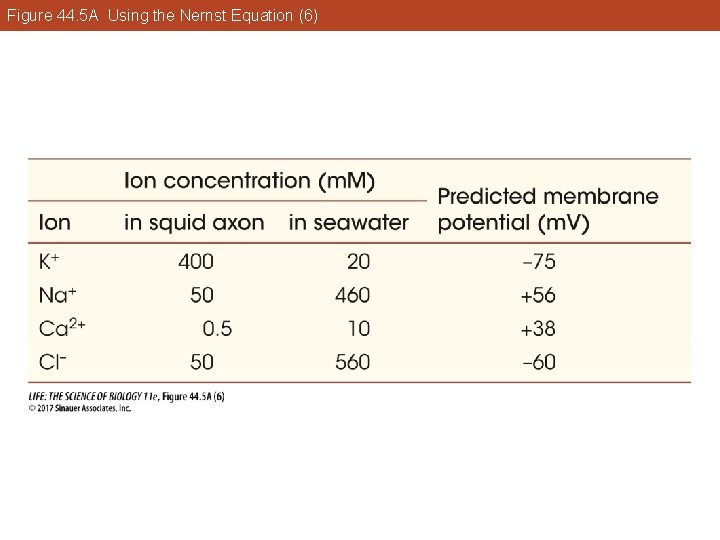
Figure 44. 5 A Using the Nernst Equation (6)
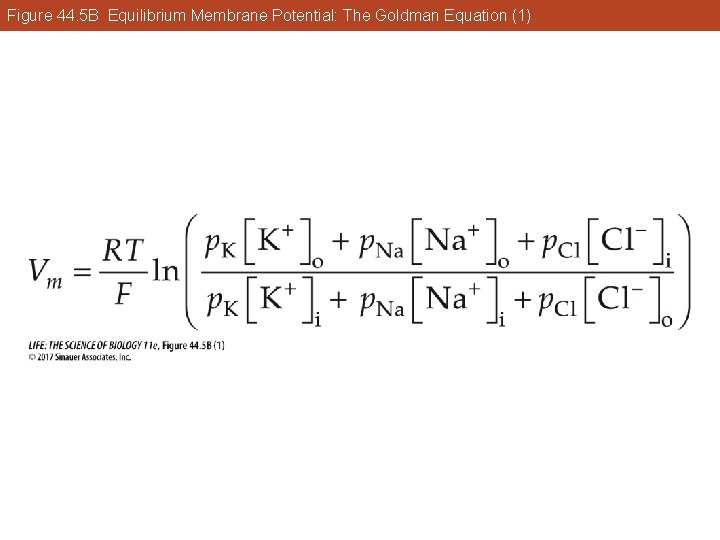
Figure 44. 5 B Equilibrium Membrane Potential: The Goldman Equation (1)
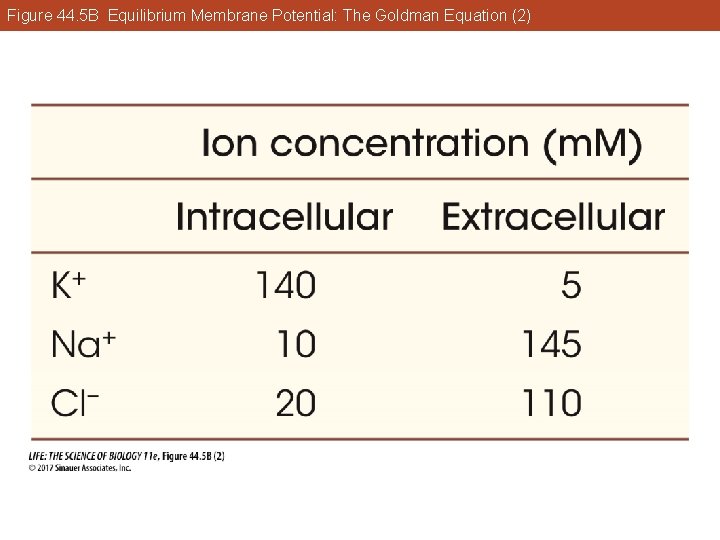
Figure 44. 5 B Equilibrium Membrane Potential: The Goldman Equation (2)
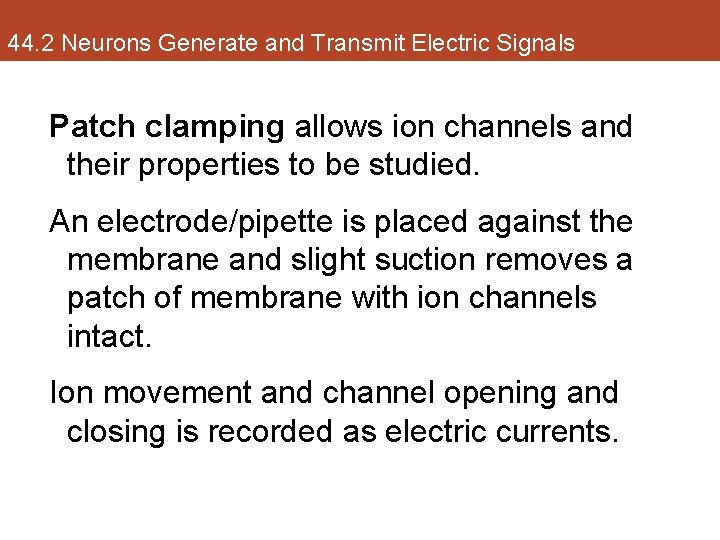
44. 2 Neurons Generate and Transmit Electric Signals Patch clamping allows ion channels and their properties to be studied. An electrode/pipette is placed against the membrane and slight suction removes a patch of membrane with ion channels intact. Ion movement and channel opening and closing is recorded as electric currents.
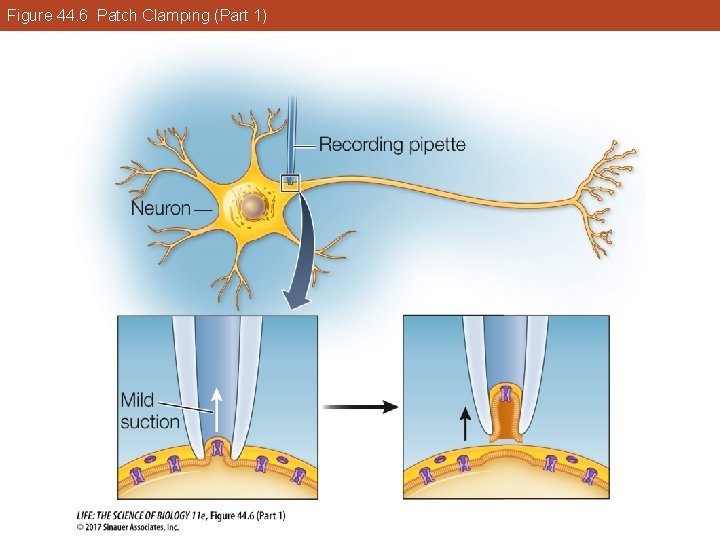
Figure 44. 6 Patch Clamping (Part 1)
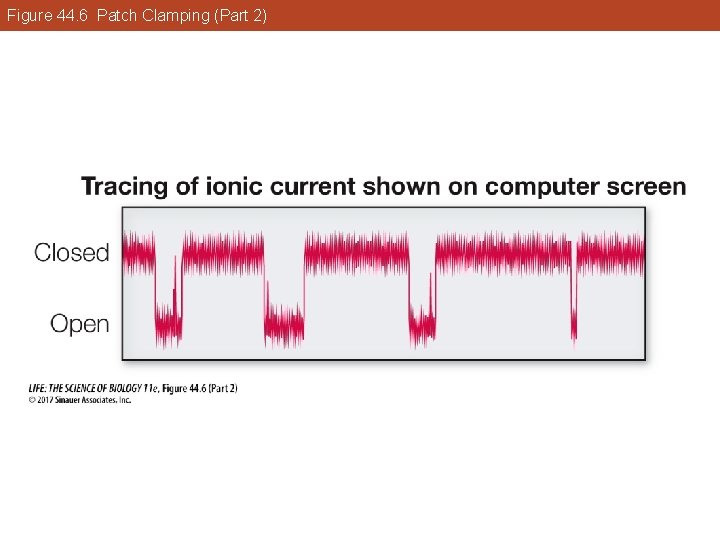
Figure 44. 6 Patch Clamping (Part 2)
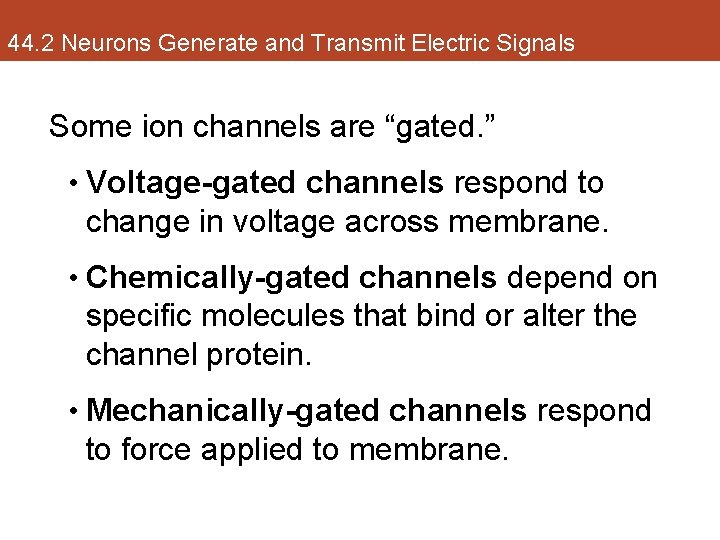
44. 2 Neurons Generate and Transmit Electric Signals Some ion channels are “gated. ” • Voltage-gated channels respond to change in voltage across membrane. • Chemically-gated channels depend on specific molecules that bind or alter the channel protein. • Mechanically-gated channels respond to force applied to membrane.
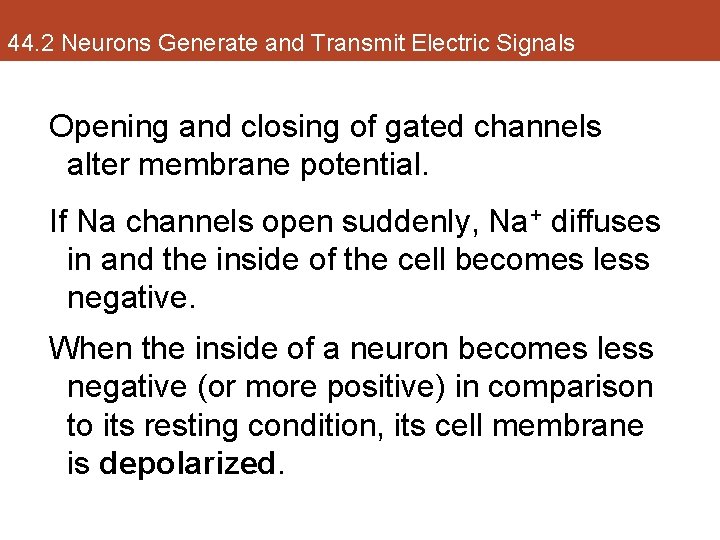
44. 2 Neurons Generate and Transmit Electric Signals Opening and closing of gated channels alter membrane potential. If Na channels open suddenly, Na+ diffuses in and the inside of the cell becomes less negative. When the inside of a neuron becomes less negative (or more positive) in comparison to its resting condition, its cell membrane is depolarized.
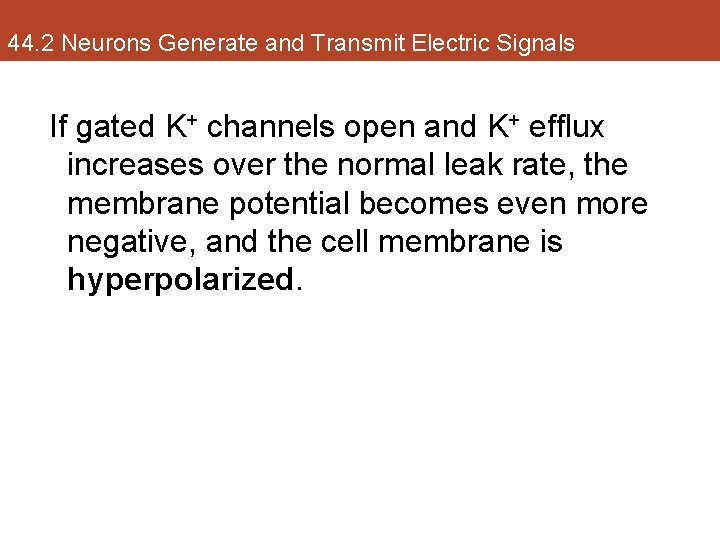
44. 2 Neurons Generate and Transmit Electric Signals If gated K+ channels open and K+ efflux increases over the normal leak rate, the membrane potential becomes even more negative, and the cell membrane is hyperpolarized.
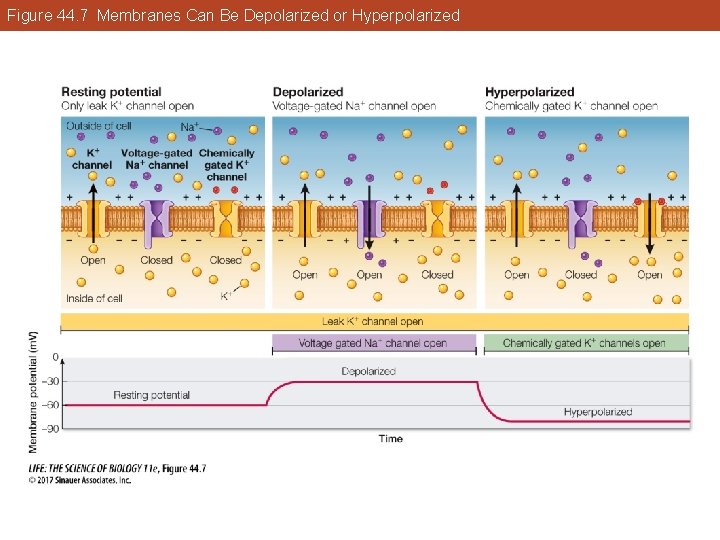
Figure 44. 7 Membranes Can Be Depolarized or Hyperpolarized
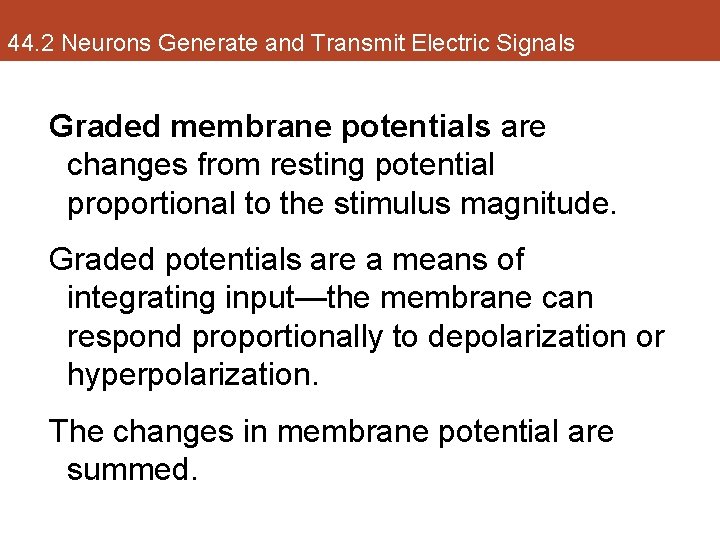
44. 2 Neurons Generate and Transmit Electric Signals Graded membrane potentials are changes from resting potential proportional to the stimulus magnitude. Graded potentials are a means of integrating input—the membrane can respond proportionally to depolarization or hyperpolarization. The changes in membrane potential are summed.
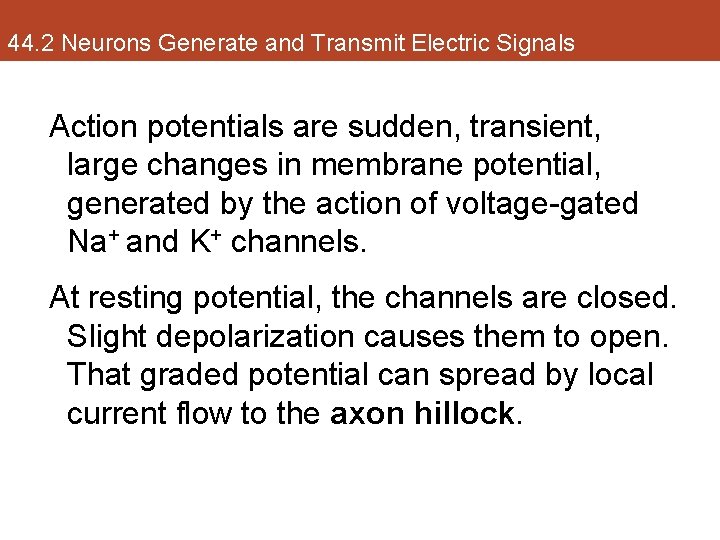
44. 2 Neurons Generate and Transmit Electric Signals Action potentials are sudden, transient, large changes in membrane potential, generated by the action of voltage-gated Na+ and K+ channels. At resting potential, the channels are closed. Slight depolarization causes them to open. That graded potential can spread by local current flow to the axon hillock.
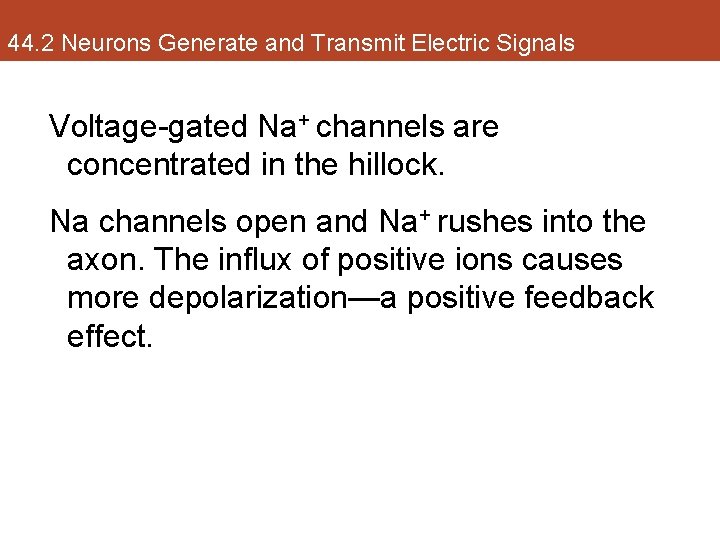
44. 2 Neurons Generate and Transmit Electric Signals Voltage-gated Na+ channels are concentrated in the hillock. Na channels open and Na+ rushes into the axon. The influx of positive ions causes more depolarization—a positive feedback effect.
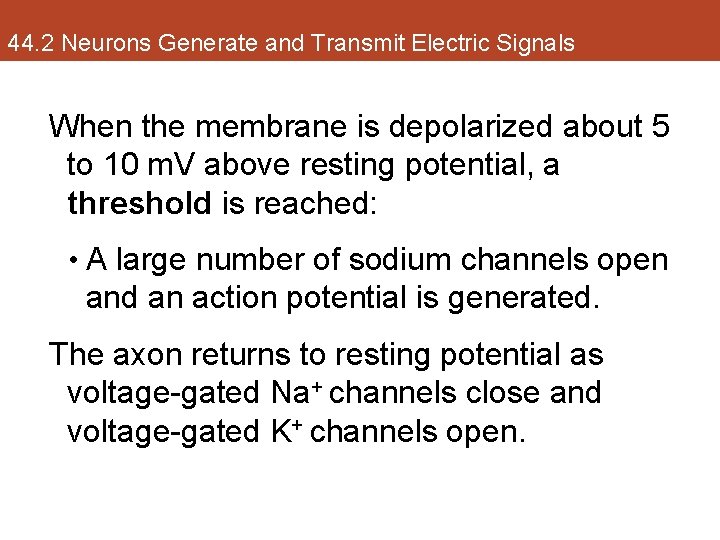
44. 2 Neurons Generate and Transmit Electric Signals When the membrane is depolarized about 5 to 10 m. V above resting potential, a threshold is reached: • A large number of sodium channels open and an action potential is generated. The axon returns to resting potential as voltage-gated Na+ channels close and voltage-gated K+ channels open.
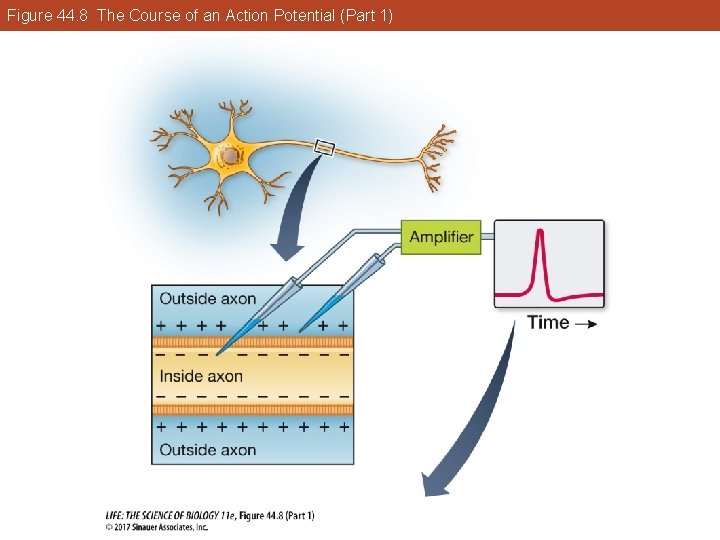
Figure 44. 8 The Course of an Action Potential (Part 1)
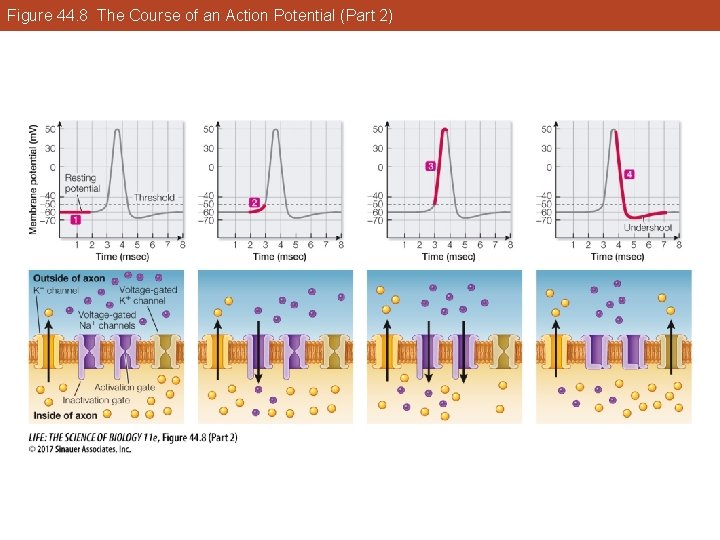
Figure 44. 8 The Course of an Action Potential (Part 2)
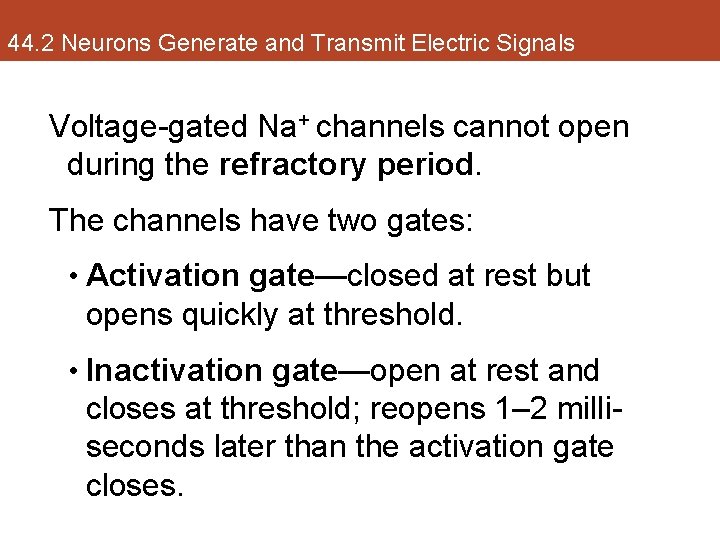
44. 2 Neurons Generate and Transmit Electric Signals Voltage-gated Na+ channels cannot open during the refractory period. The channels have two gates: • Activation gate—closed at rest but opens quickly at threshold. • Inactivation gate—open at rest and closes at threshold; reopens 1– 2 milliseconds later than the activation gate closes.
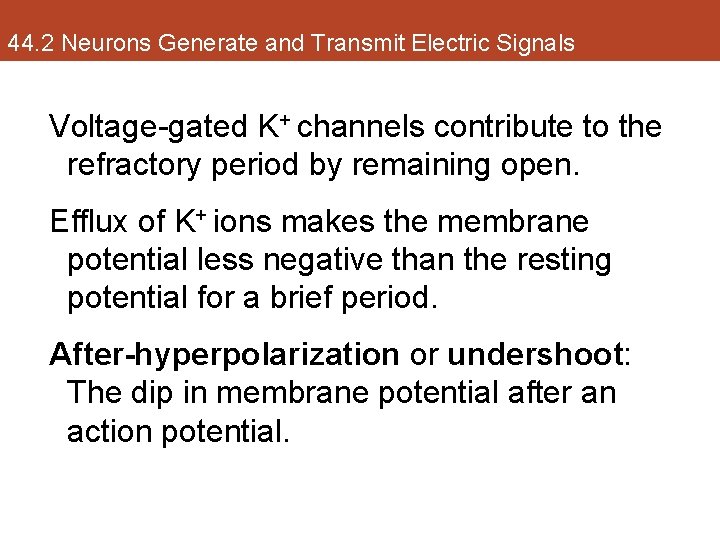
44. 2 Neurons Generate and Transmit Electric Signals Voltage-gated K+ channels contribute to the refractory period by remaining open. Efflux of K+ ions makes the membrane potential less negative than the resting potential for a brief period. After-hyperpolarization or undershoot: The dip in membrane potential after an action potential.
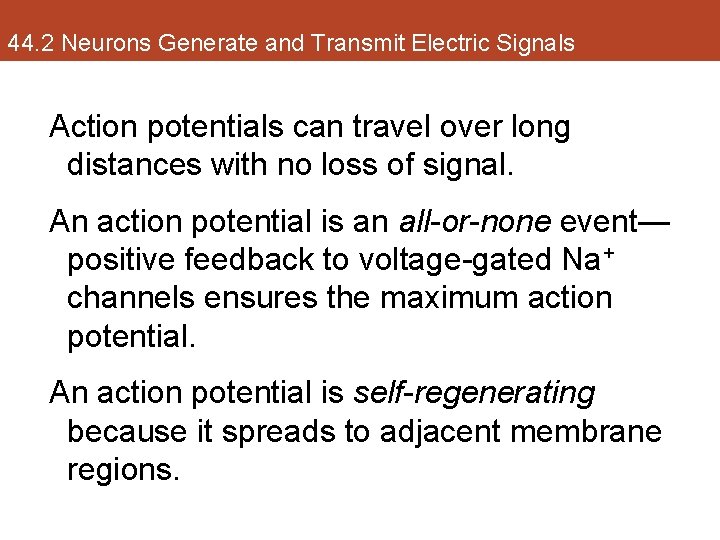
44. 2 Neurons Generate and Transmit Electric Signals Action potentials can travel over long distances with no loss of signal. An action potential is an all-or-none event— positive feedback to voltage-gated Na+ channels ensures the maximum action potential. An action potential is self-regenerating because it spreads to adjacent membrane regions.
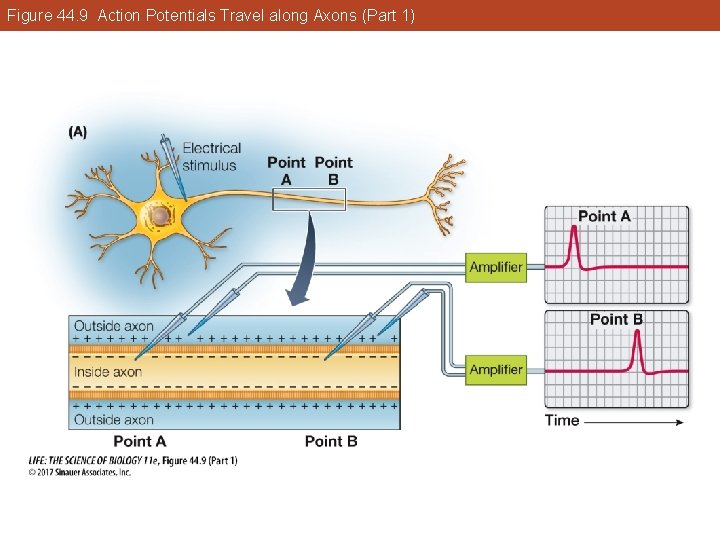
Figure 44. 9 Action Potentials Travel along Axons (Part 1)
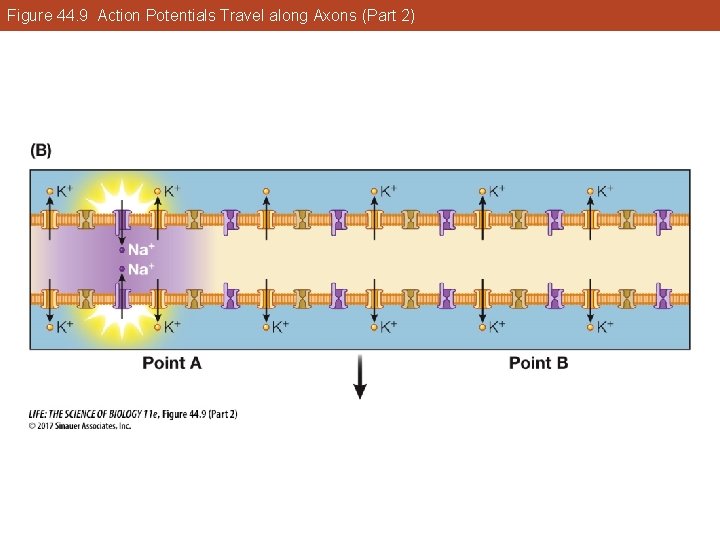
Figure 44. 9 Action Potentials Travel along Axons (Part 2)
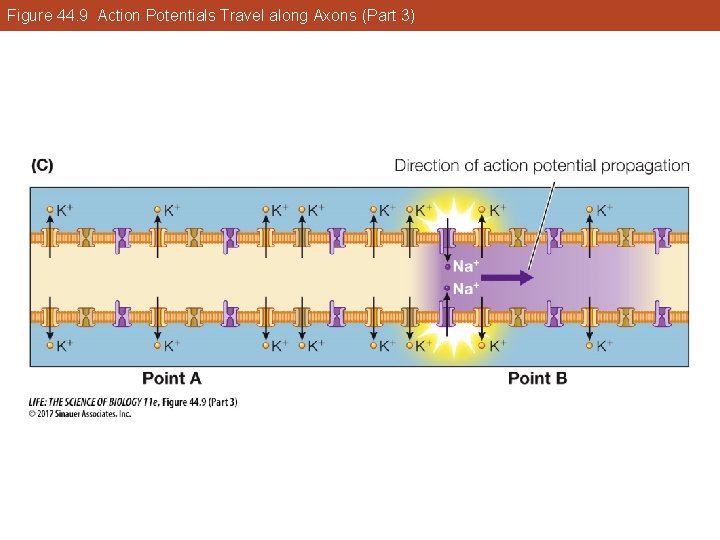
Figure 44. 9 Action Potentials Travel along Axons (Part 3)
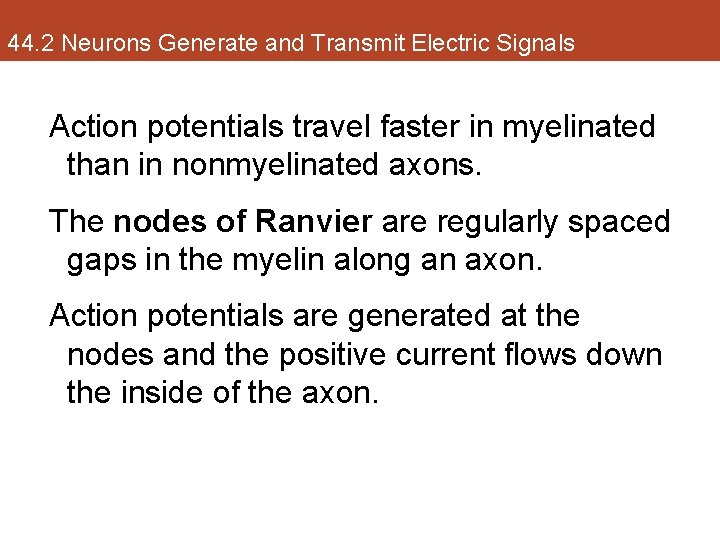
44. 2 Neurons Generate and Transmit Electric Signals Action potentials travel faster in myelinated than in nonmyelinated axons. The nodes of Ranvier are regularly spaced gaps in the myelin along an axon. Action potentials are generated at the nodes and the positive current flows down the inside of the axon.
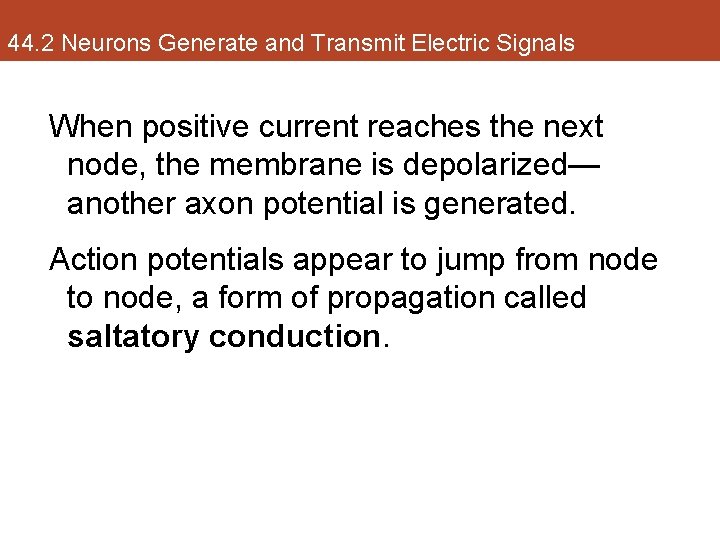
44. 2 Neurons Generate and Transmit Electric Signals When positive current reaches the next node, the membrane is depolarized— another axon potential is generated. Action potentials appear to jump from node to node, a form of propagation called saltatory conduction.
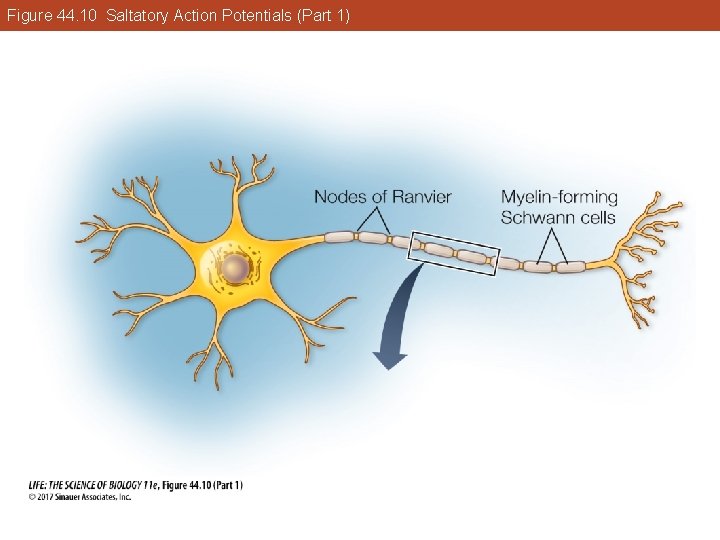
Figure 44. 10 Saltatory Action Potentials (Part 1)
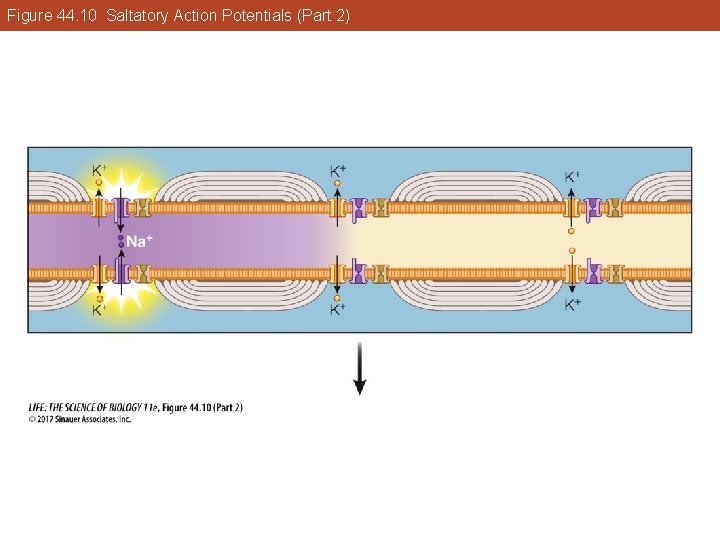
Figure 44. 10 Saltatory Action Potentials (Part 2)
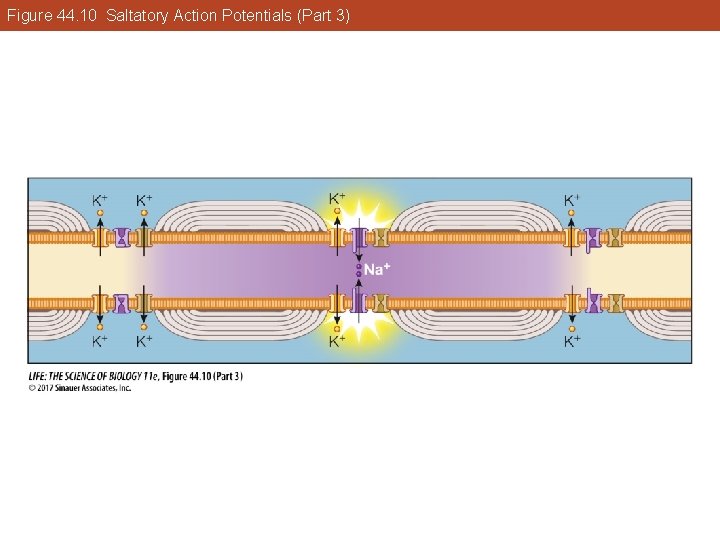
Figure 44. 10 Saltatory Action Potentials (Part 3)
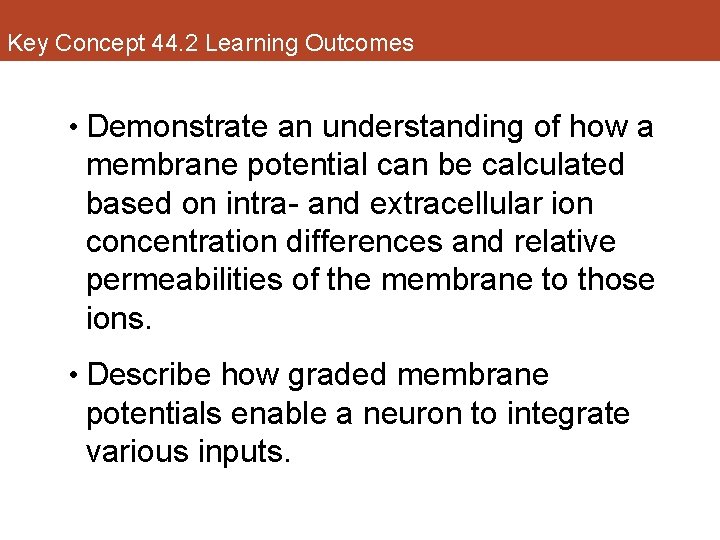
Key Concept 44. 2 Learning Outcomes • Demonstrate an understanding of how a membrane potential can be calculated based on intra- and extracellular ion concentration differences and relative permeabilities of the membrane to those ions. • Describe how graded membrane potentials enable a neuron to integrate various inputs.
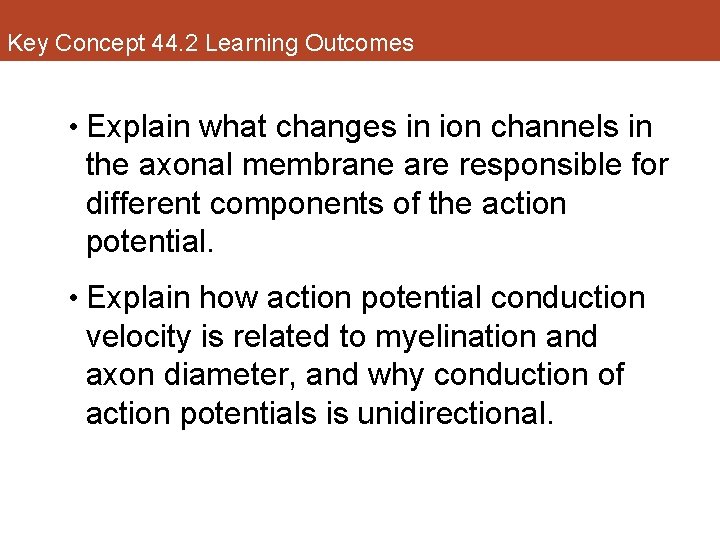
Key Concept 44. 2 Learning Outcomes • Explain what changes in ion channels in the axonal membrane are responsible for different components of the action potential. • Explain how action potential conduction velocity is related to myelination and axon diameter, and why conduction of action potentials is unidirectional.
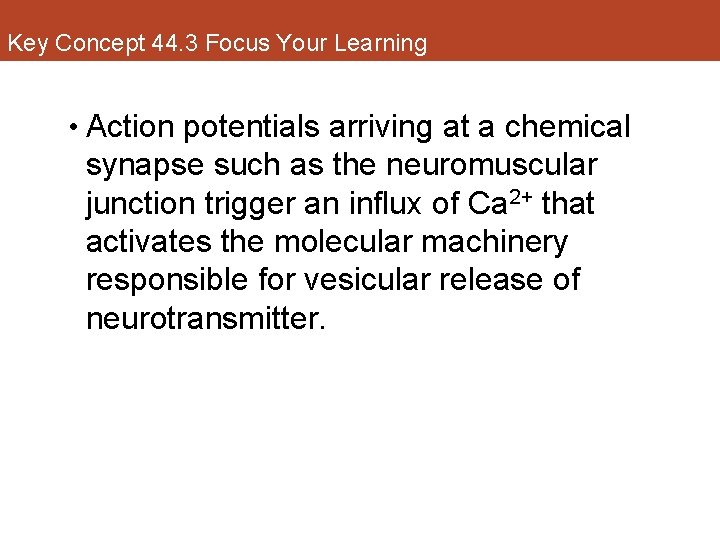
Key Concept 44. 3 Focus Your Learning • Action potentials arriving at a chemical synapse such as the neuromuscular junction trigger an influx of Ca 2+ that activates the molecular machinery responsible for vesicular release of neurotransmitter.
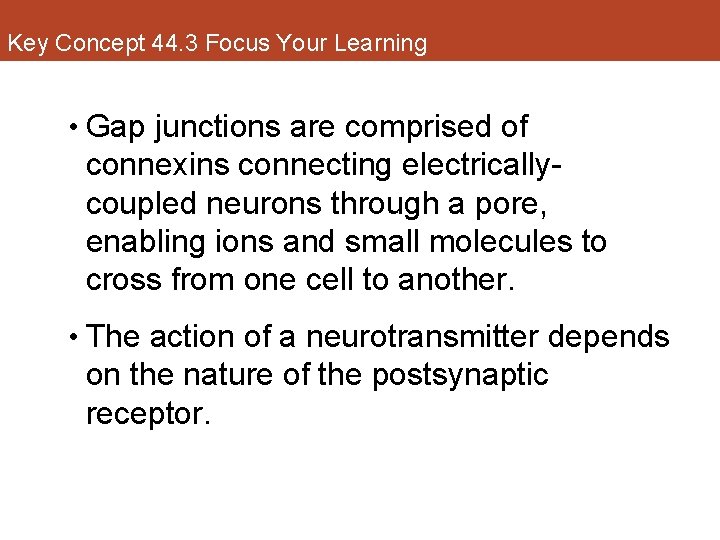
Key Concept 44. 3 Focus Your Learning • Gap junctions are comprised of connexins connecting electricallycoupled neurons through a pore, enabling ions and small molecules to cross from one cell to another. • The action of a neurotransmitter depends on the nature of the postsynaptic receptor.
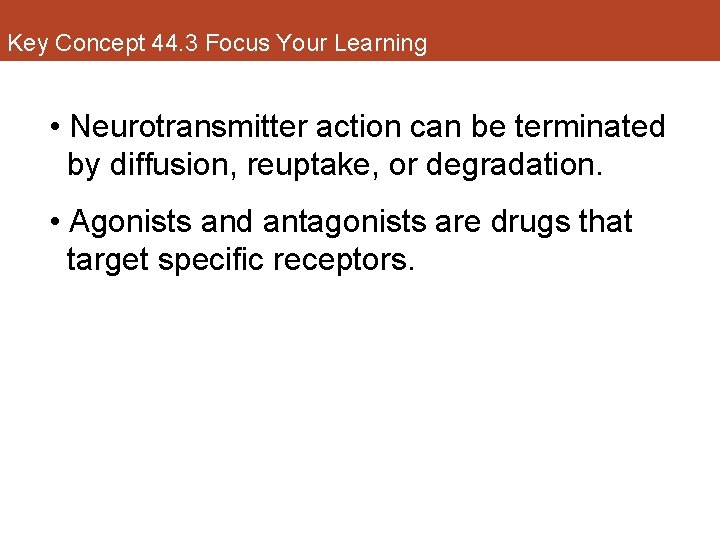
Key Concept 44. 3 Focus Your Learning • Neurotransmitter action can be terminated by diffusion, reuptake, or degradation. • Agonists and antagonists are drugs that target specific receptors.
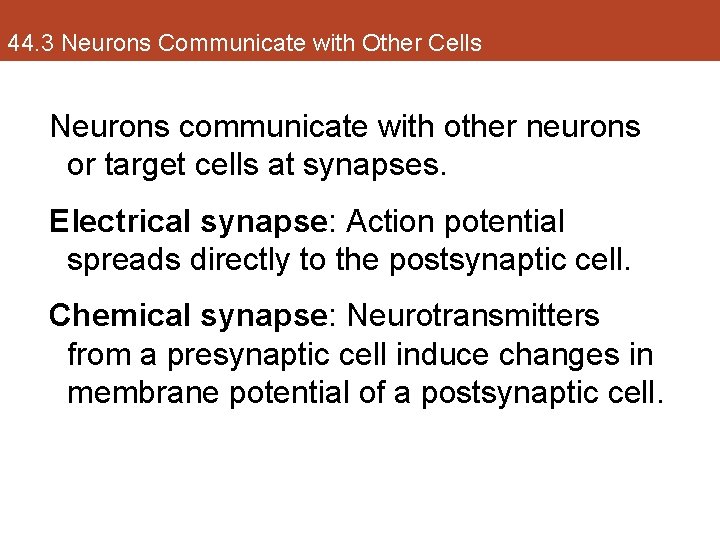
44. 3 Neurons Communicate with Other Cells Neurons communicate with other neurons or target cells at synapses. Electrical synapse: Action potential spreads directly to the postsynaptic cell. Chemical synapse: Neurotransmitters from a presynaptic cell induce changes in membrane potential of a postsynaptic cell.
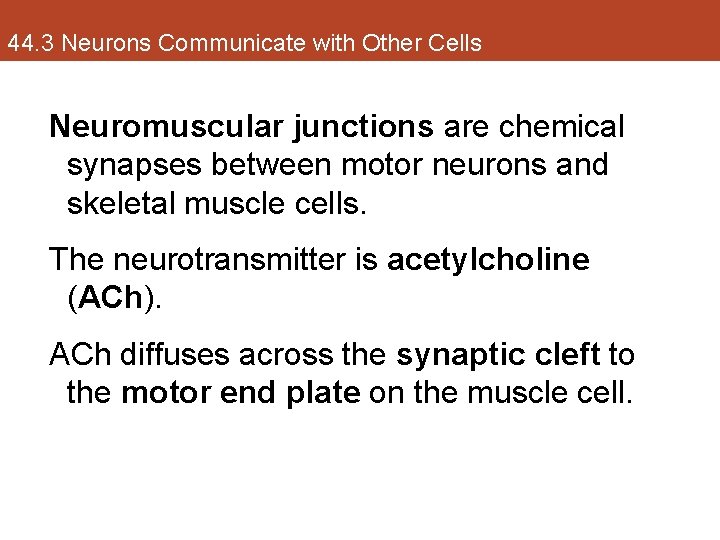
44. 3 Neurons Communicate with Other Cells Neuromuscular junctions are chemical synapses between motor neurons and skeletal muscle cells. The neurotransmitter is acetylcholine (ACh). ACh diffuses across the synaptic cleft to the motor end plate on the muscle cell.
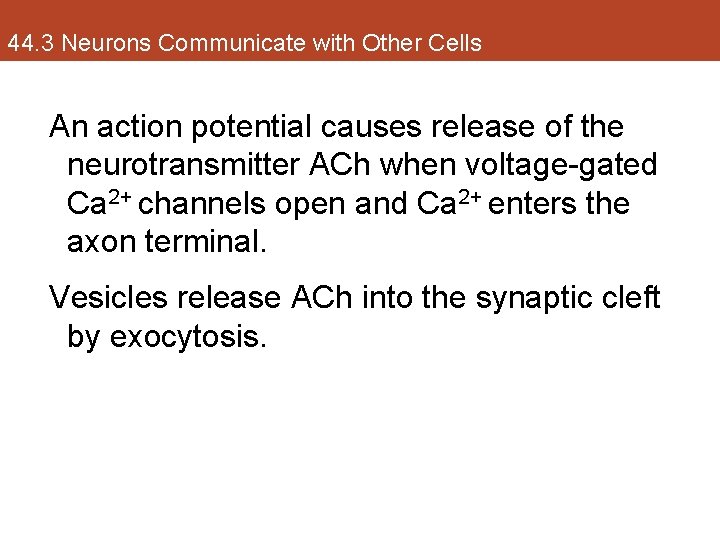
44. 3 Neurons Communicate with Other Cells An action potential causes release of the neurotransmitter ACh when voltage-gated Ca 2+ channels open and Ca 2+ enters the axon terminal. Vesicles release ACh into the synaptic cleft by exocytosis.
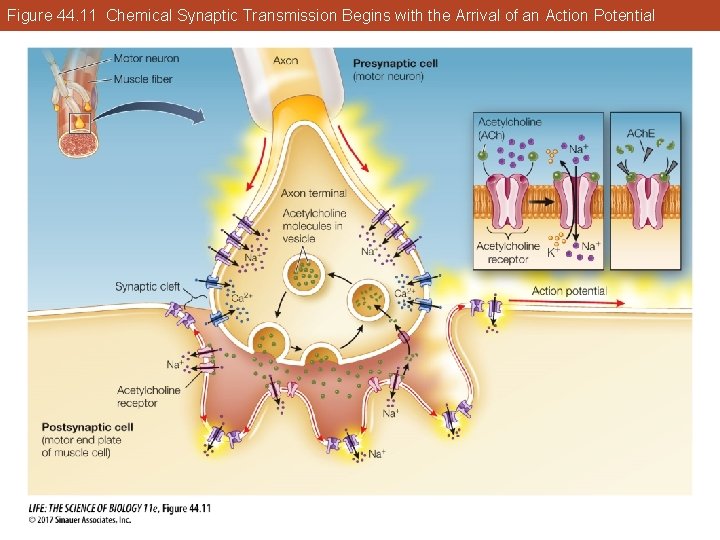
Figure 44. 11 Chemical Synaptic Transmission Begins with the Arrival of an Action Potential
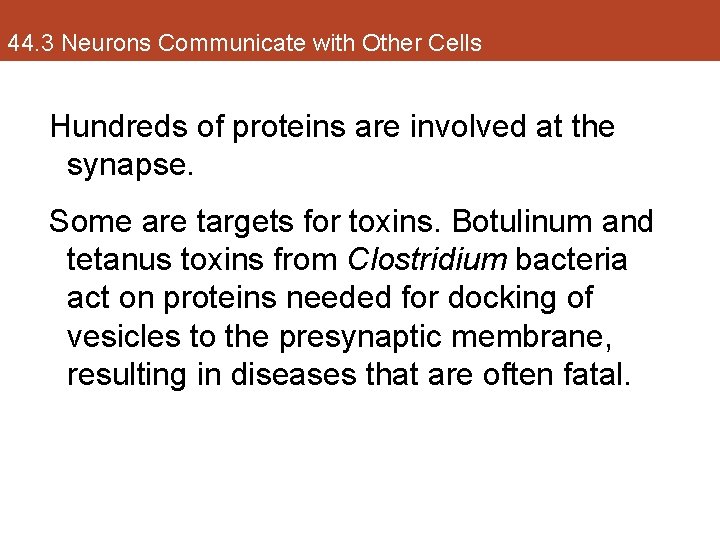
44. 3 Neurons Communicate with Other Cells Hundreds of proteins are involved at the synapse. Some are targets for toxins. Botulinum and tetanus toxins from Clostridium bacteria act on proteins needed for docking of vesicles to the presynaptic membrane, resulting in diseases that are often fatal.
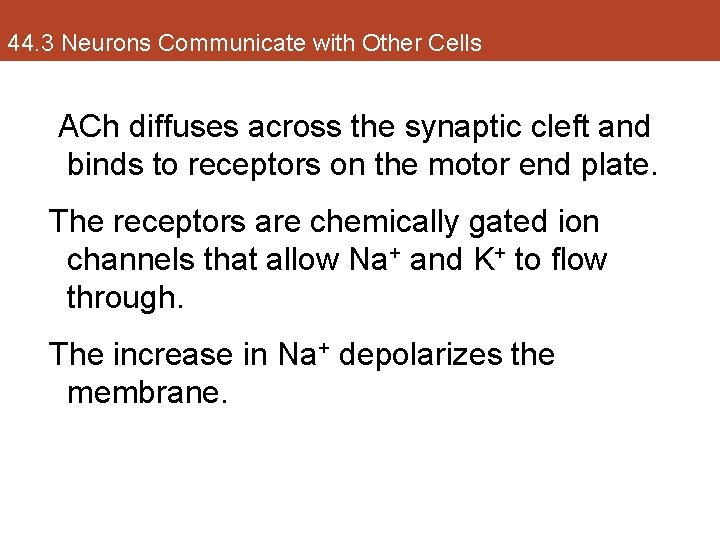
44. 3 Neurons Communicate with Other Cells ACh diffuses across the synaptic cleft and binds to receptors on the motor end plate. The receptors are chemically gated ion channels that allow Na+ and K+ to flow through. The increase in Na+ depolarizes the membrane.
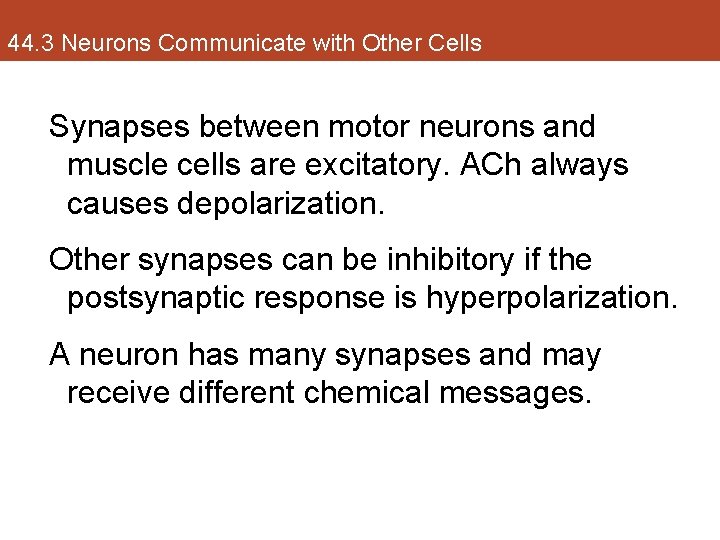
44. 3 Neurons Communicate with Other Cells Synapses between motor neurons and muscle cells are excitatory. ACh always causes depolarization. Other synapses can be inhibitory if the postsynaptic response is hyperpolarization. A neuron has many synapses and may receive different chemical messages.
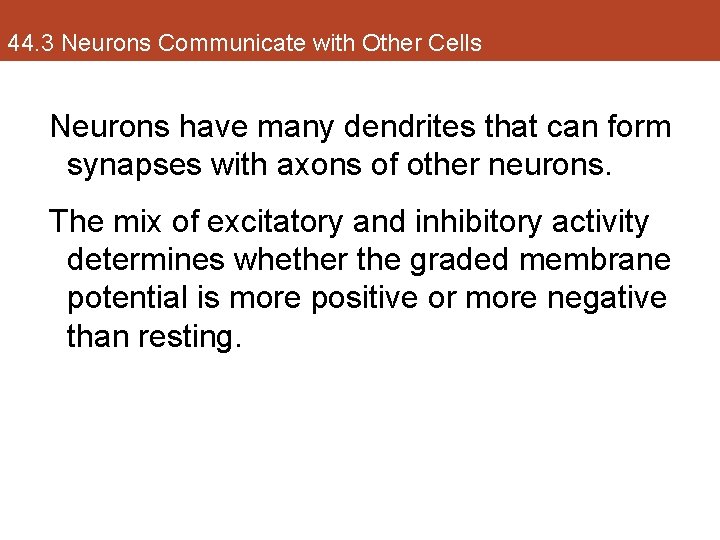
44. 3 Neurons Communicate with Other Cells Neurons have many dendrites that can form synapses with axons of other neurons. The mix of excitatory and inhibitory activity determines whether the graded membrane potential is more positive or more negative than resting.
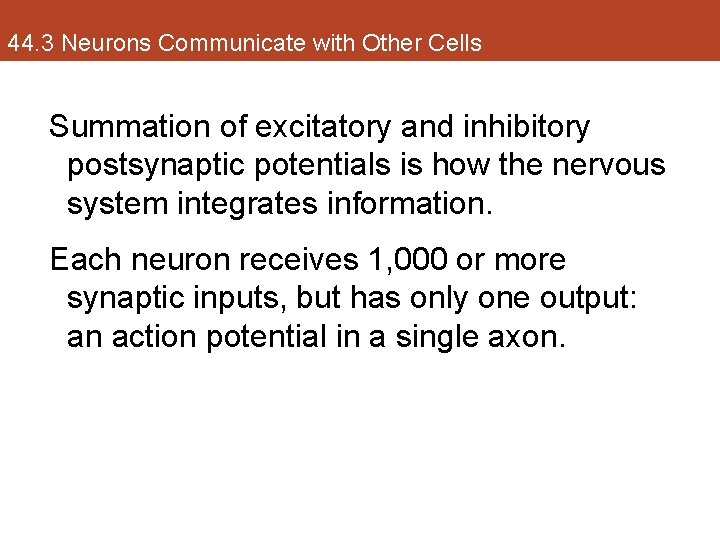
44. 3 Neurons Communicate with Other Cells Summation of excitatory and inhibitory postsynaptic potentials is how the nervous system integrates information. Each neuron receives 1, 000 or more synaptic inputs, but has only one output: an action potential in a single axon.
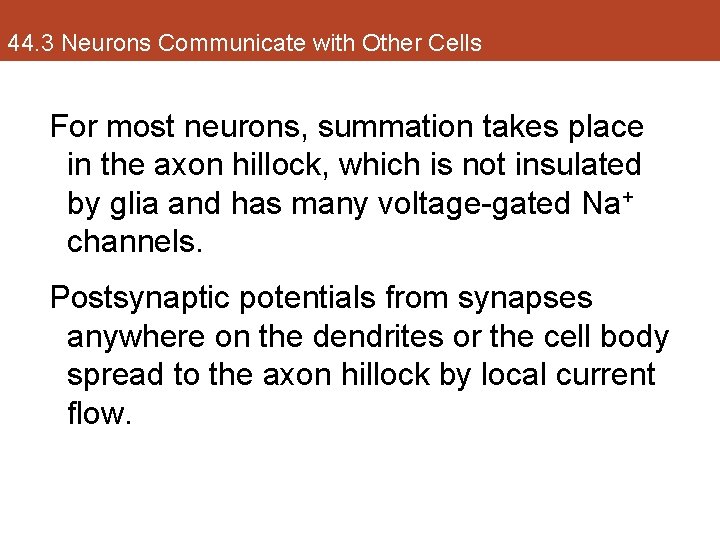
44. 3 Neurons Communicate with Other Cells For most neurons, summation takes place in the axon hillock, which is not insulated by glia and has many voltage-gated Na+ channels. Postsynaptic potentials from synapses anywhere on the dendrites or the cell body spread to the axon hillock by local current flow.
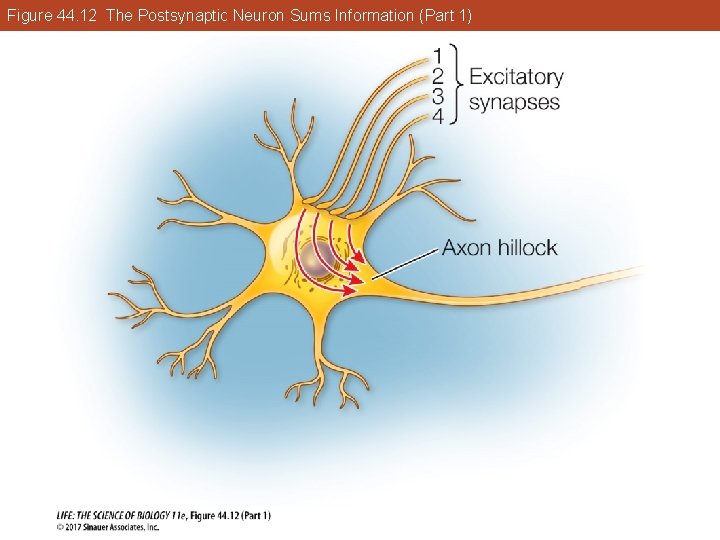
Figure 44. 12 The Postsynaptic Neuron Sums Information (Part 1)
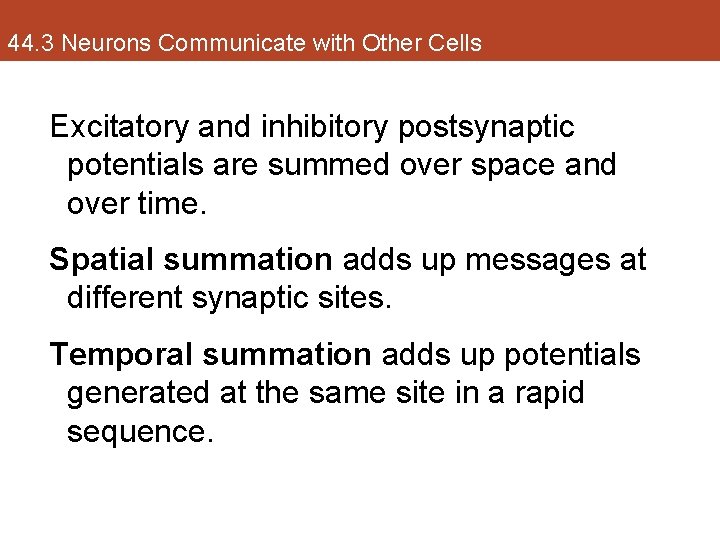
44. 3 Neurons Communicate with Other Cells Excitatory and inhibitory postsynaptic potentials are summed over space and over time. Spatial summation adds up messages at different synaptic sites. Temporal summation adds up potentials generated at the same site in a rapid sequence.
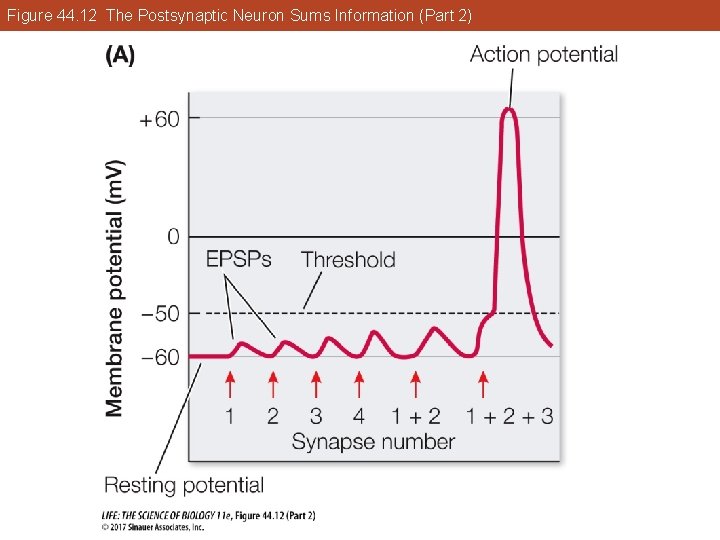
Figure 44. 12 The Postsynaptic Neuron Sums Information (Part 2)
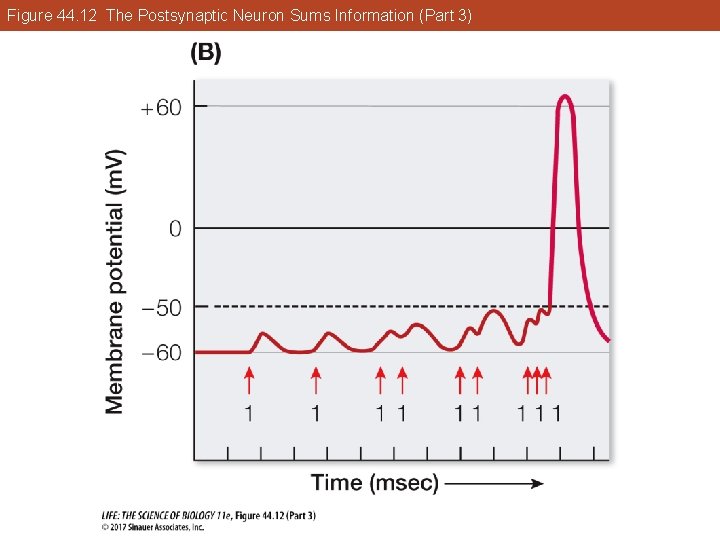
Figure 44. 12 The Postsynaptic Neuron Sums Information (Part 3)
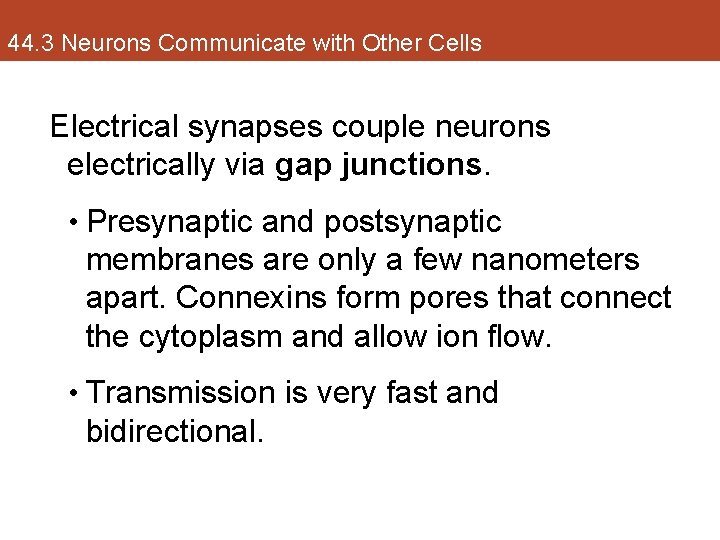
44. 3 Neurons Communicate with Other Cells Electrical synapses couple neurons electrically via gap junctions. • Presynaptic and postsynaptic membranes are only a few nanometers apart. Connexins form pores that connect the cytoplasm and allow ion flow. • Transmission is very fast and bidirectional.
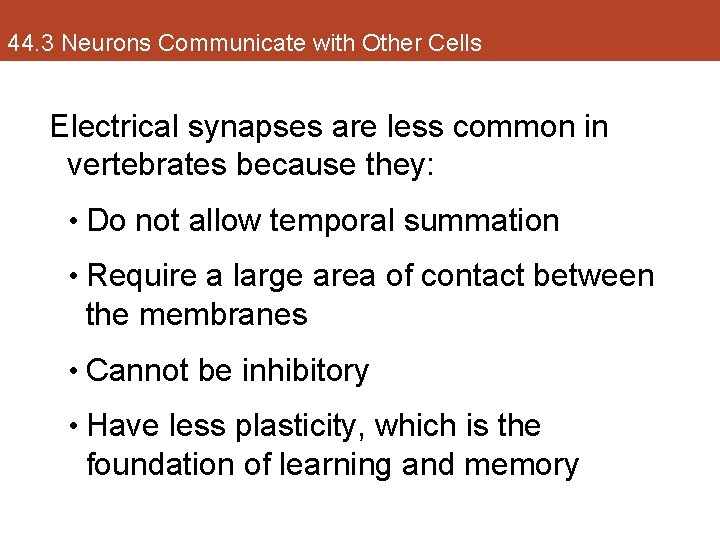
44. 3 Neurons Communicate with Other Cells Electrical synapses are less common in vertebrates because they: • Do not allow temporal summation • Require a large area of contact between the membranes • Cannot be inhibitory • Have less plasticity, which is the foundation of learning and memory
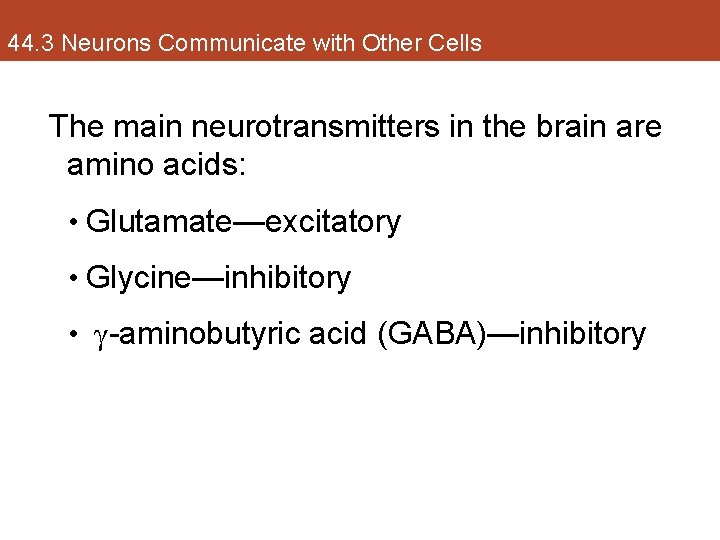
44. 3 Neurons Communicate with Other Cells The main neurotransmitters in the brain are amino acids: • Glutamate—excitatory • Glycine—inhibitory • γ-aminobutyric acid (GABA)—inhibitory
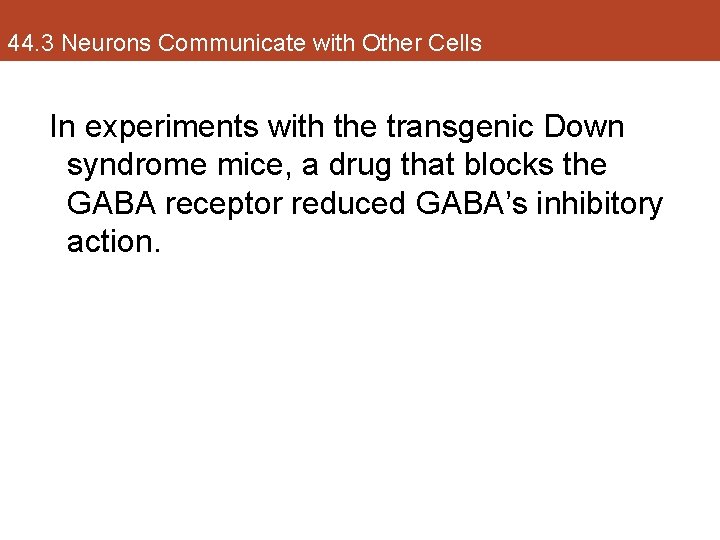
44. 3 Neurons Communicate with Other Cells In experiments with the transgenic Down syndrome mice, a drug that blocks the GABA receptor reduced GABA’s inhibitory action.
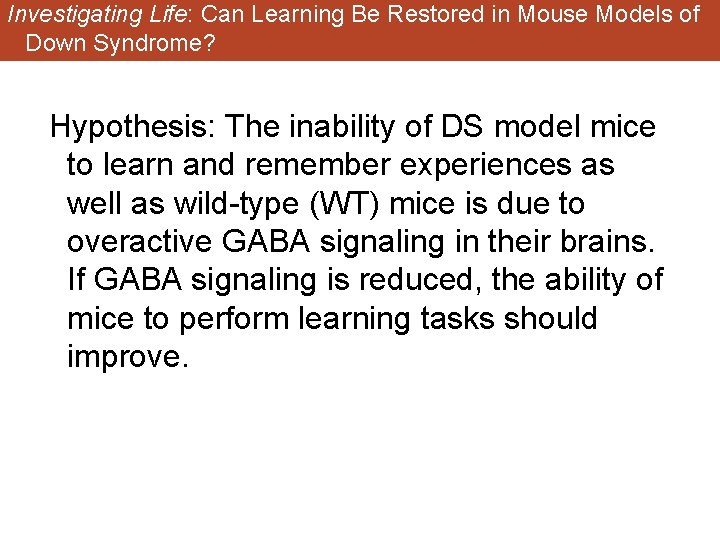
Investigating Life: Can Learning Be Restored in Mouse Models of Down Syndrome? Hypothesis: The inability of DS model mice to learn and remember experiences as well as wild-type (WT) mice is due to overactive GABA signaling in their brains. If GABA signaling is reduced, the ability of mice to perform learning tasks should improve.
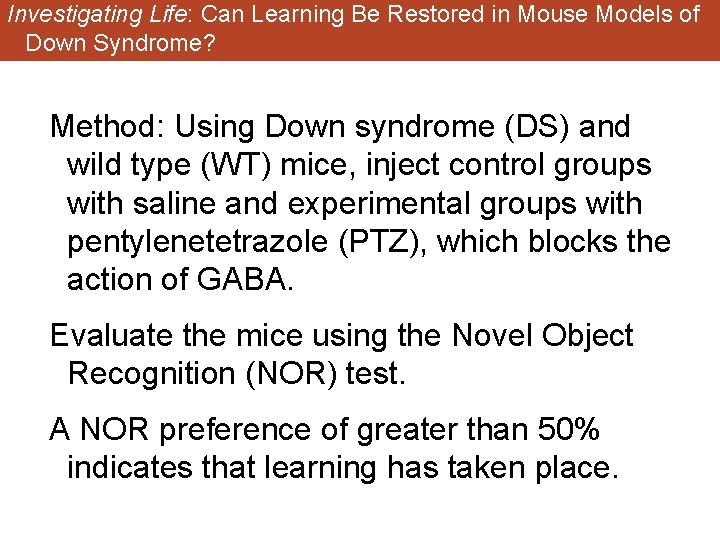
Investigating Life: Can Learning Be Restored in Mouse Models of Down Syndrome? Method: Using Down syndrome (DS) and wild type (WT) mice, inject control groups with saline and experimental groups with pentylenetetrazole (PTZ), which blocks the action of GABA. Evaluate the mice using the Novel Object Recognition (NOR) test. A NOR preference of greater than 50% indicates that learning has taken place.
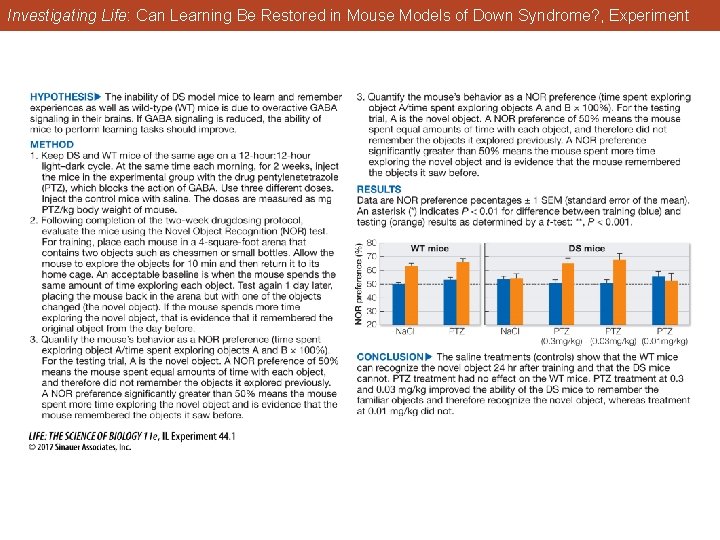
Investigating Life: Can Learning Be Restored in Mouse Models of Down Syndrome? , Experiment
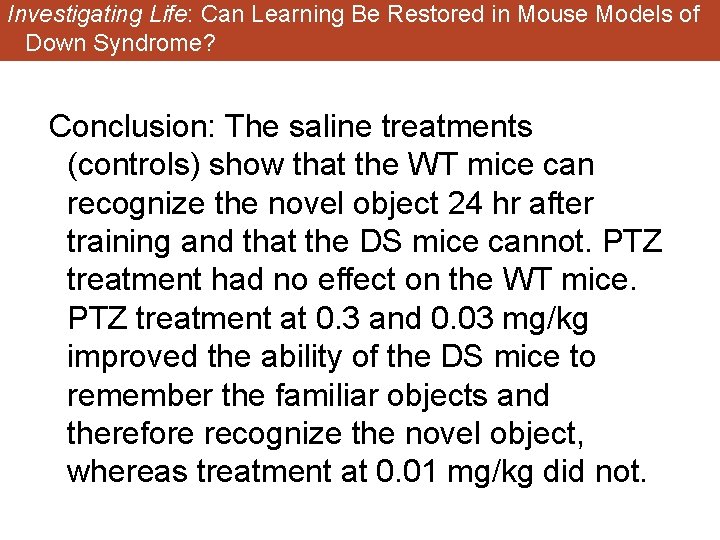
Investigating Life: Can Learning Be Restored in Mouse Models of Down Syndrome? Conclusion: The saline treatments (controls) show that the WT mice can recognize the novel object 24 hr after training and that the DS mice cannot. PTZ treatment had no effect on the WT mice. PTZ treatment at 0. 3 and 0. 03 mg/kg improved the ability of the DS mice to remember the familiar objects and therefore recognize the novel object, whereas treatment at 0. 01 mg/kg did not.
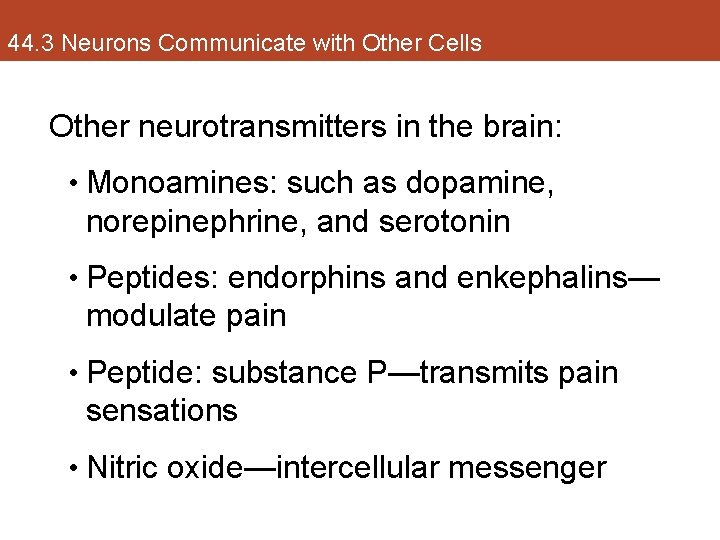
44. 3 Neurons Communicate with Other Cells Other neurotransmitters in the brain: • Monoamines: such as dopamine, norepinephrine, and serotonin • Peptides: endorphins and enkephalins— modulate pain • Peptide: substance P—transmits pain sensations • Nitric oxide—intercellular messenger
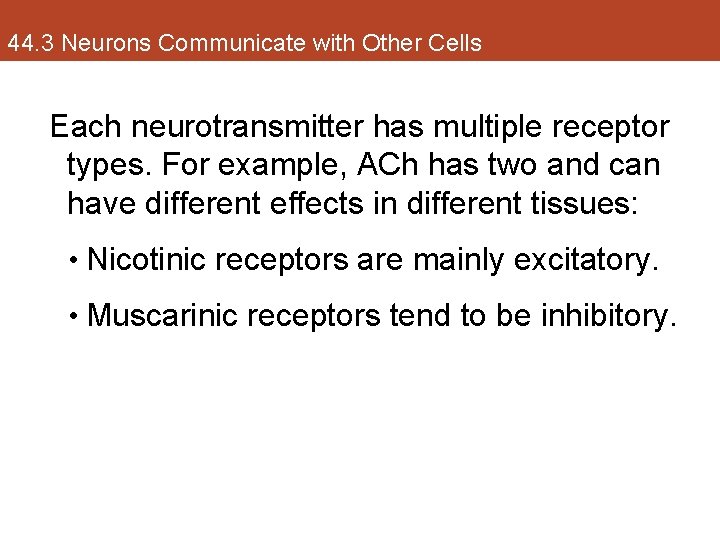
44. 3 Neurons Communicate with Other Cells Each neurotransmitter has multiple receptor types. For example, ACh has two and can have different effects in different tissues: • Nicotinic receptors are mainly excitatory. • Muscarinic receptors tend to be inhibitory.
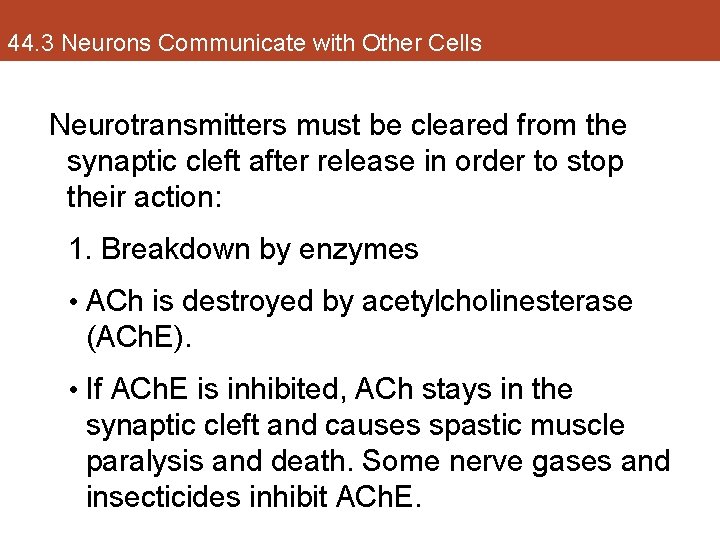
44. 3 Neurons Communicate with Other Cells Neurotransmitters must be cleared from the synaptic cleft after release in order to stop their action: 1. Breakdown by enzymes • ACh is destroyed by acetylcholinesterase (ACh. E). • If ACh. E is inhibited, ACh stays in the synaptic cleft and causes spastic muscle paralysis and death. Some nerve gases and insecticides inhibit ACh. E.
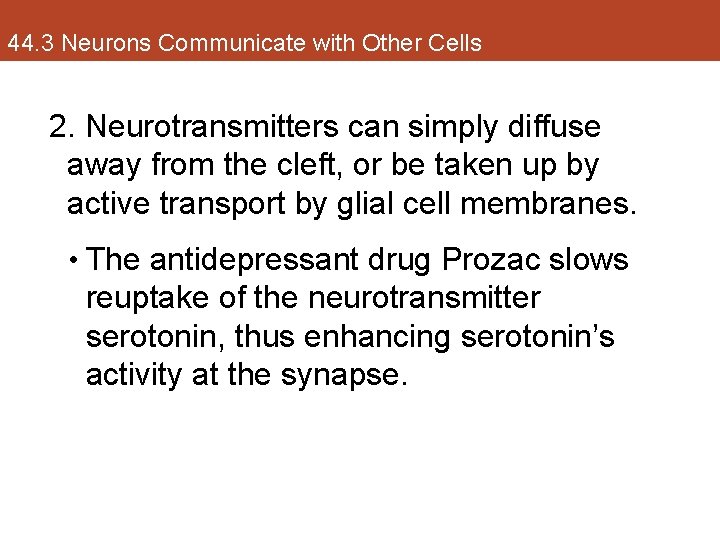
44. 3 Neurons Communicate with Other Cells 2. Neurotransmitters can simply diffuse away from the cleft, or be taken up by active transport by glial cell membranes. • The antidepressant drug Prozac slows reuptake of the neurotransmitter serotonin, thus enhancing serotonin’s activity at the synapse.
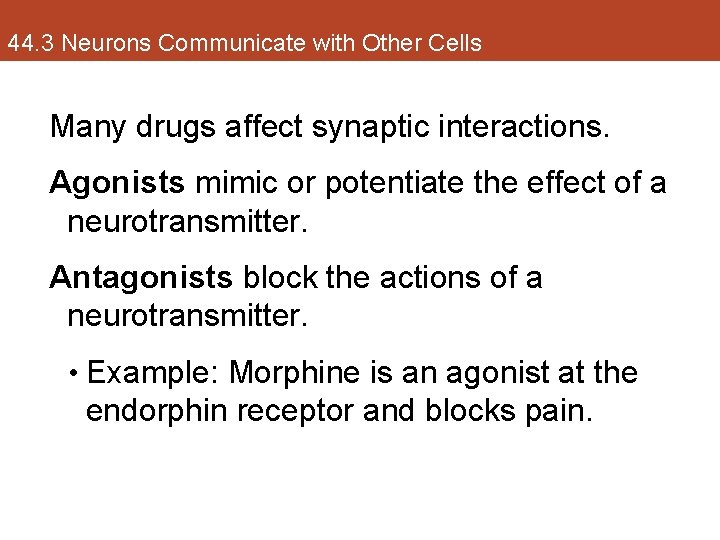
44. 3 Neurons Communicate with Other Cells Many drugs affect synaptic interactions. Agonists mimic or potentiate the effect of a neurotransmitter. Antagonists block the actions of a neurotransmitter. • Example: Morphine is an agonist at the endorphin receptor and blocks pain.
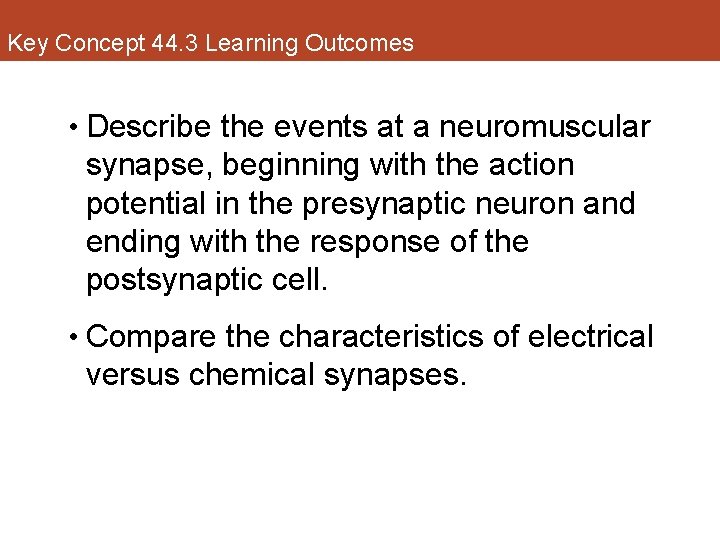
Key Concept 44. 3 Learning Outcomes • Describe the events at a neuromuscular synapse, beginning with the action potential in the presynaptic neuron and ending with the response of the postsynaptic cell. • Compare the characteristics of electrical versus chemical synapses.
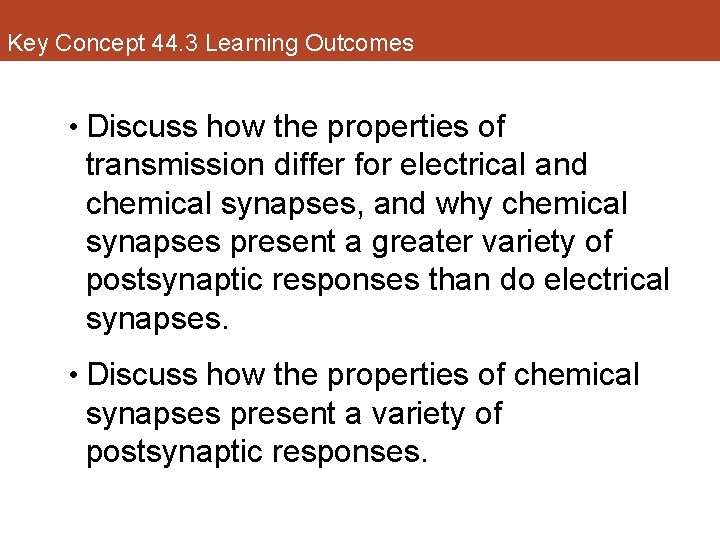
Key Concept 44. 3 Learning Outcomes • Discuss how the properties of transmission differ for electrical and chemical synapses, and why chemical synapses present a greater variety of postsynaptic responses than do electrical synapses. • Discuss how the properties of chemical synapses present a variety of postsynaptic responses.
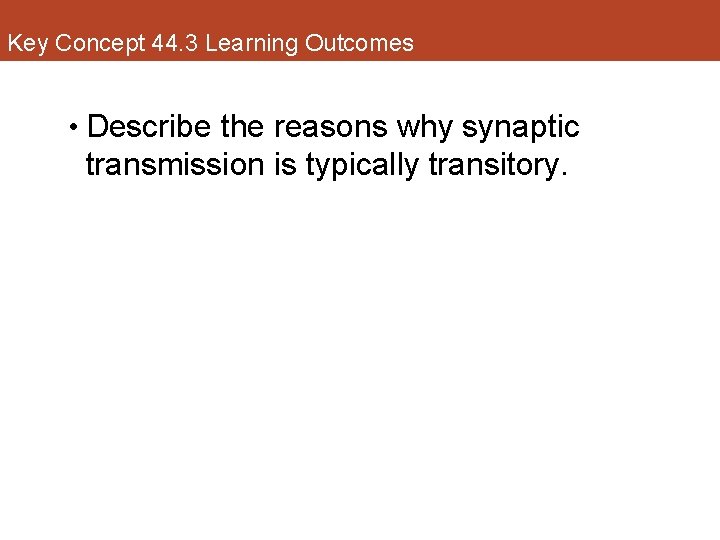
Key Concept 44. 3 Learning Outcomes • Describe the reasons why synaptic transmission is typically transitory.
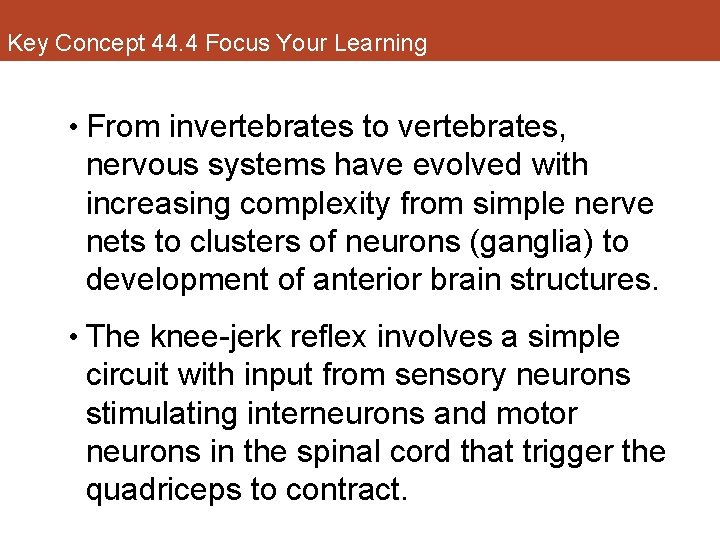
Key Concept 44. 4 Focus Your Learning • From invertebrates to vertebrates, nervous systems have evolved with increasing complexity from simple nerve nets to clusters of neurons (ganglia) to development of anterior brain structures. • The knee-jerk reflex involves a simple circuit with input from sensory neurons stimulating interneurons and motor neurons in the spinal cord that trigger the quadriceps to contract.
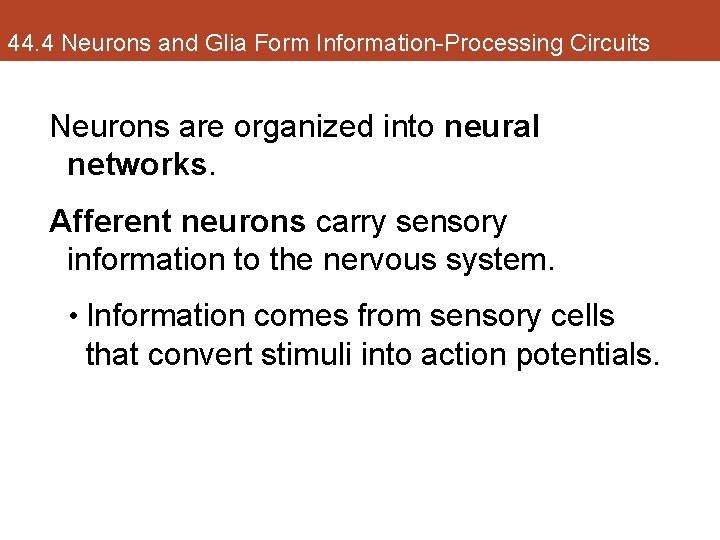
44. 4 Neurons and Glia Form Information-Processing Circuits Neurons are organized into neural networks. Afferent neurons carry sensory information to the nervous system. • Information comes from sensory cells that convert stimuli into action potentials.
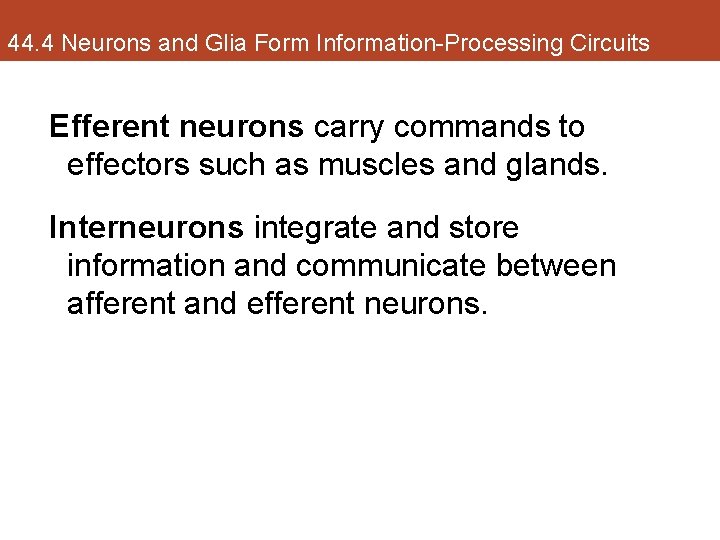
44. 4 Neurons and Glia Form Information-Processing Circuits Efferent neurons carry commands to effectors such as muscles and glands. Interneurons integrate and store information and communicate between afferent and efferent neurons.
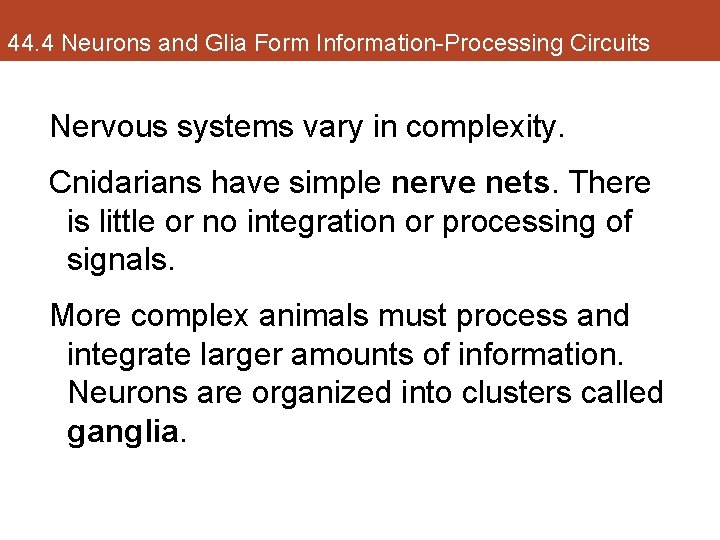
44. 4 Neurons and Glia Form Information-Processing Circuits Nervous systems vary in complexity. Cnidarians have simple nerve nets. There is little or no integration or processing of signals. More complex animals must process and integrate larger amounts of information. Neurons are organized into clusters called ganglia.
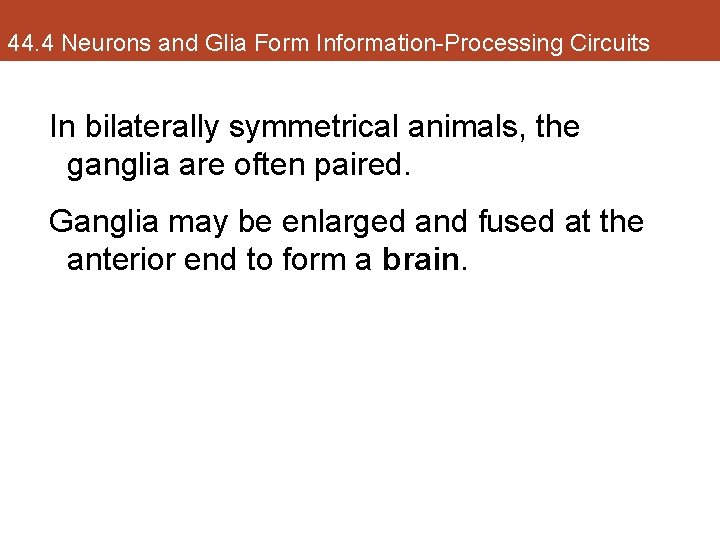
44. 4 Neurons and Glia Form Information-Processing Circuits In bilaterally symmetrical animals, the ganglia are often paired. Ganglia may be enlarged and fused at the anterior end to form a brain.
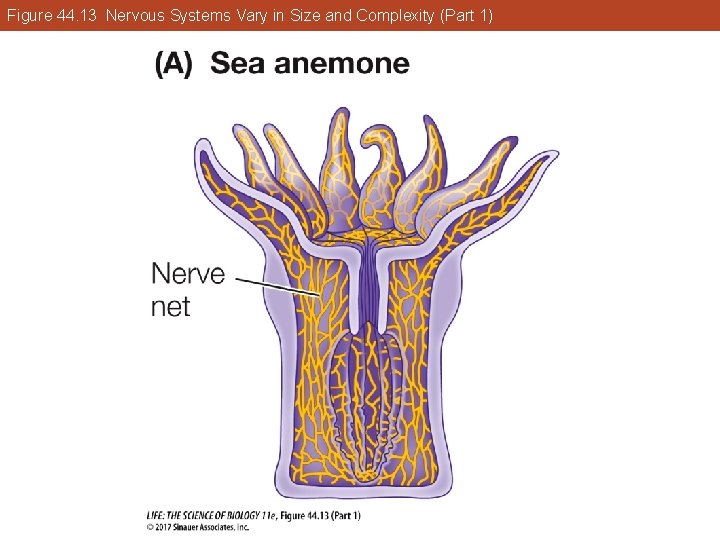
Figure 44. 13 Nervous Systems Vary in Size and Complexity (Part 1)
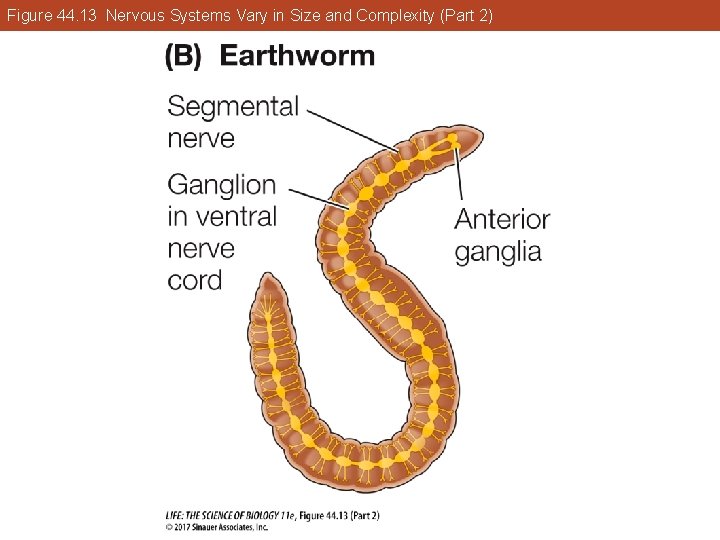
Figure 44. 13 Nervous Systems Vary in Size and Complexity (Part 2)
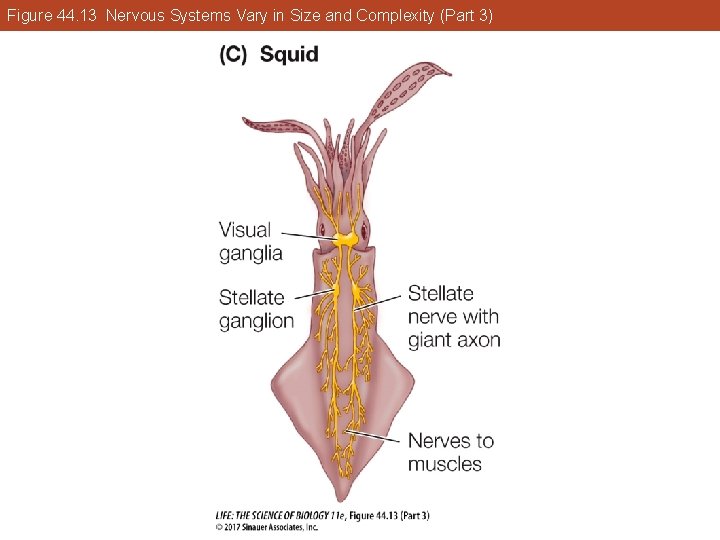
Figure 44. 13 Nervous Systems Vary in Size and Complexity (Part 3)
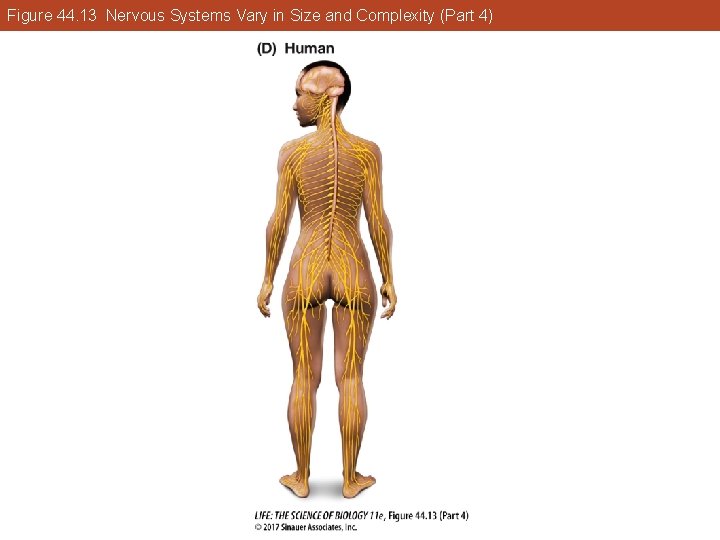
Figure 44. 13 Nervous Systems Vary in Size and Complexity (Part 4)
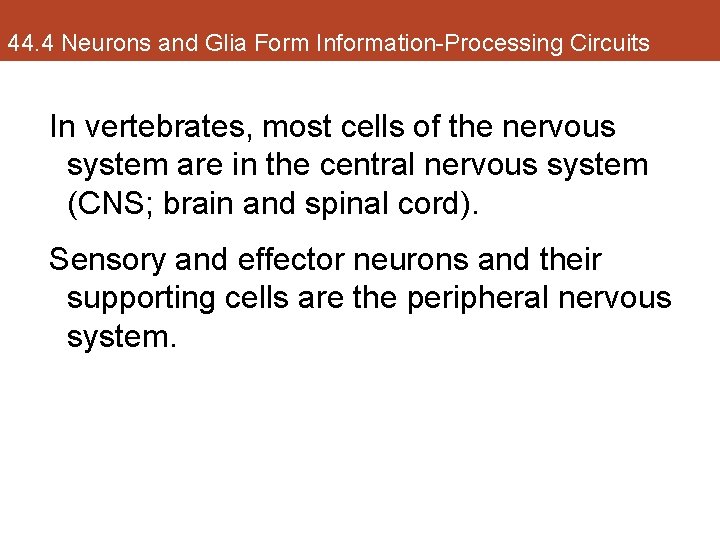
44. 4 Neurons and Glia Form Information-Processing Circuits In vertebrates, most cells of the nervous system are in the central nervous system (CNS; brain and spinal cord). Sensory and effector neurons and their supporting cells are the peripheral nervous system.
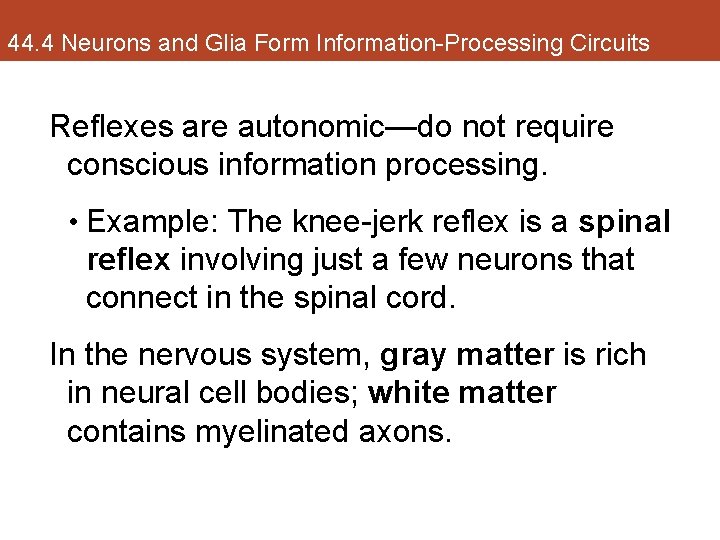
44. 4 Neurons and Glia Form Information-Processing Circuits Reflexes are autonomic—do not require conscious information processing. • Example: The knee-jerk reflex is a spinal reflex involving just a few neurons that connect in the spinal cord. In the nervous system, gray matter is rich in neural cell bodies; white matter contains myelinated axons.
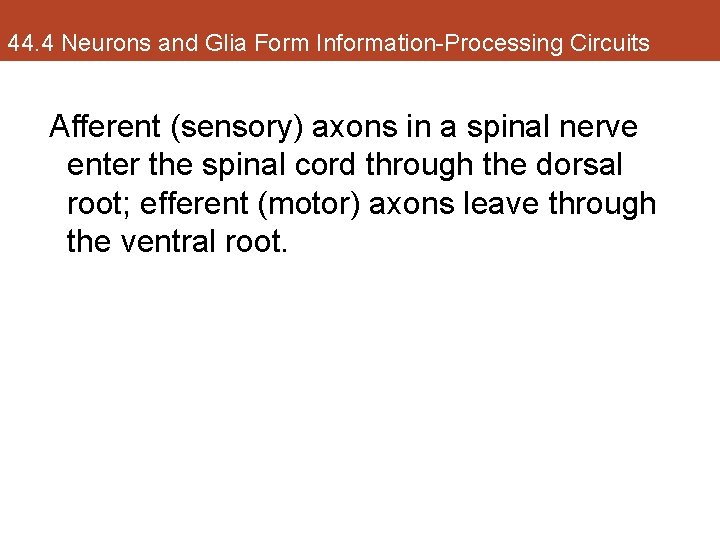
44. 4 Neurons and Glia Form Information-Processing Circuits Afferent (sensory) axons in a spinal nerve enter the spinal cord through the dorsal root; efferent (motor) axons leave through the ventral root.
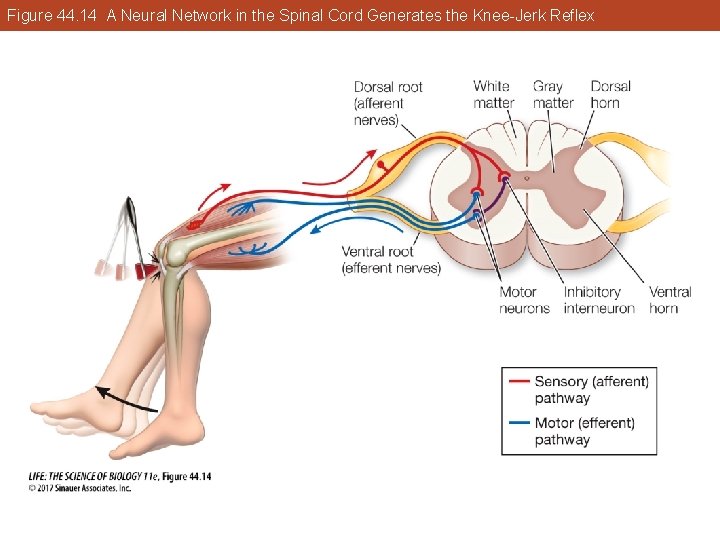
Figure 44. 14 A Neural Network in the Spinal Cord Generates the Knee-Jerk Reflex
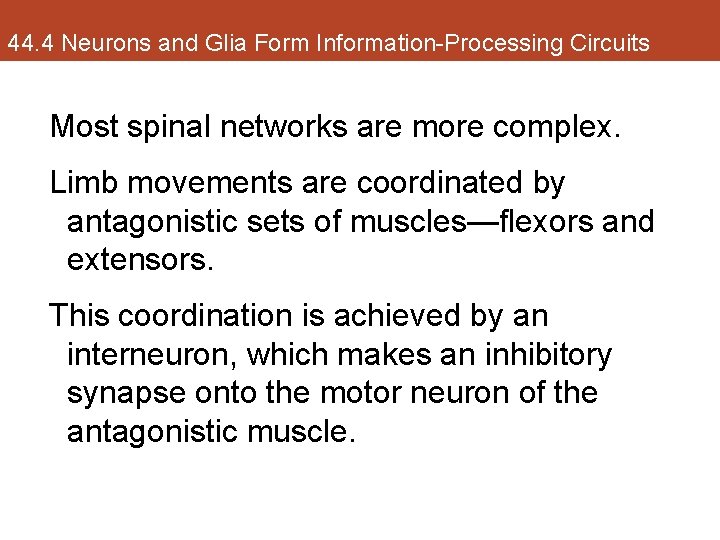
44. 4 Neurons and Glia Form Information-Processing Circuits Most spinal networks are more complex. Limb movements are coordinated by antagonistic sets of muscles—flexors and extensors. This coordination is achieved by an interneuron, which makes an inhibitory synapse onto the motor neuron of the antagonistic muscle.
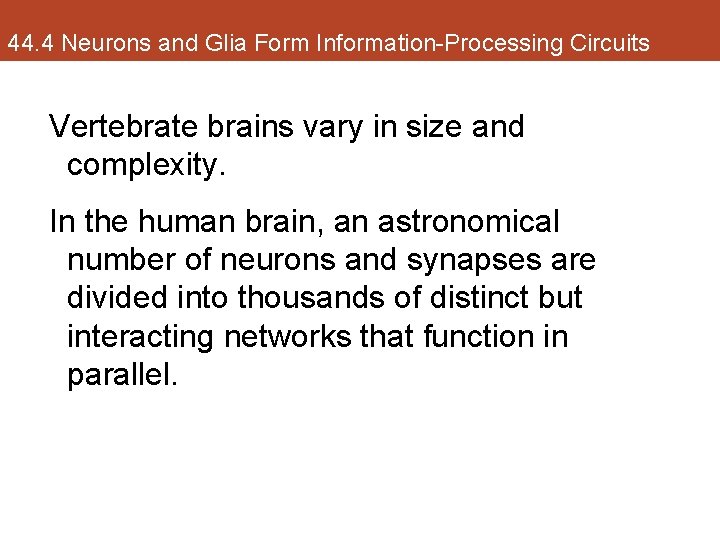
44. 4 Neurons and Glia Form Information-Processing Circuits Vertebrate brains vary in size and complexity. In the human brain, an astronomical number of neurons and synapses are divided into thousands of distinct but interacting networks that function in parallel.
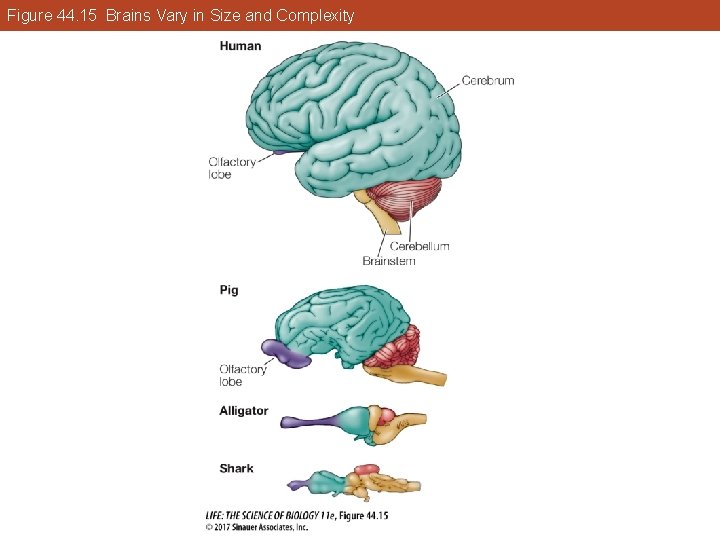
Figure 44. 15 Brains Vary in Size and Complexity
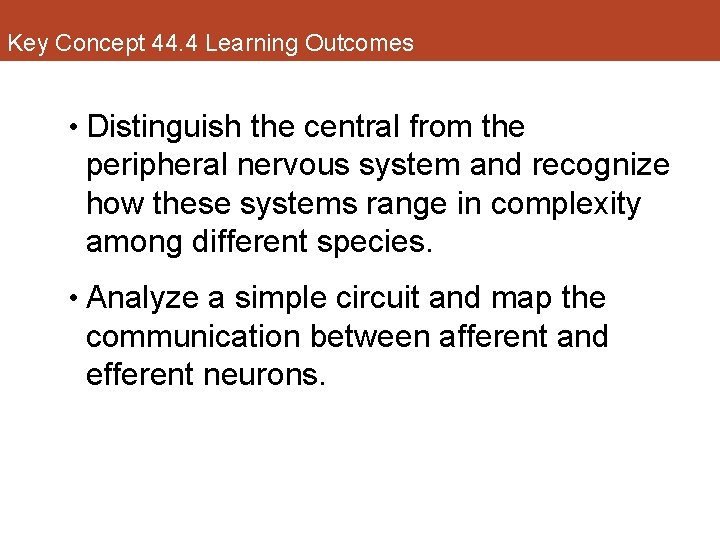
Key Concept 44. 4 Learning Outcomes • Distinguish the central from the peripheral nervous system and recognize how these systems range in complexity among different species. • Analyze a simple circuit and map the communication between afferent and efferent neurons.
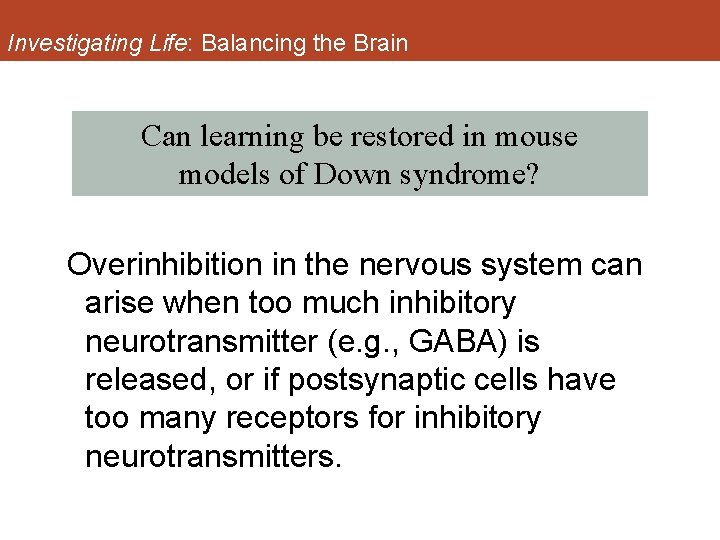
Investigating Life: Balancing the Brain Can learning be restored in mouse models of Down syndrome? Overinhibition in the nervous system can arise when too much inhibitory neurotransmitter (e. g. , GABA) is released, or if postsynaptic cells have too many receptors for inhibitory neurotransmitters.
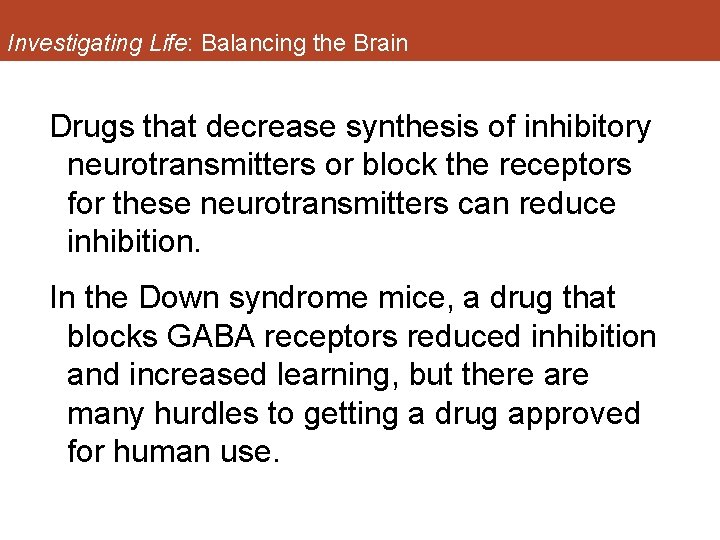
Investigating Life: Balancing the Brain Drugs that decrease synthesis of inhibitory neurotransmitters or block the receptors for these neurotransmitters can reduce inhibition. In the Down syndrome mice, a drug that blocks GABA receptors reduced inhibition and increased learning, but there are many hurdles to getting a drug approved for human use.
Neuronal pools
Fundamentals of the nervous system and nervous tissue
Processes neuron
Schede didattiche con gla gle gli glo glu
Chapter 14 the skeletal muscular and nervous systems
Differences between nervous system and endocrine
Prevertebral ganglia
Divisions of the nervous system
Nervous interactions with other systems
Type 1 cutaneous mechanoreceptors
Somatic motor association area
What is the stretch reflex
Pyramidal pathway
Autonomic vs somatic
Figure 8-2 neurons and neuroglia
Chapter 48 neurons synapses and signaling
Chapter 48 neurons synapses and signaling
Virtual neurons
Brain homunculus
Via optica
Divergence neurons
Input neurons
Proprioception autism
Mirror neurons location
Input neurons
Label the different types of neuronal pools in the figure.
Fourth order neurons
Vestibulocochlear nerve nuclei
Mirror neurons ap psych
Characteristics of sensory neurons
What are neurons composed of
Nervous system communication
Efferent motor neurons
Unipolar neurons are found in
Information flow through neurons
How neurons communicate
Sensory modality examples
Biopsychology revision
Secondary reinforcer ap psychology definition
Unipolar neurons are found in
Upper motor neurons
Neurons
Central and peripheral nervous system
Sensory neuron
Pruning neurons
Resting membrane potential of neurons
Language
Mirror neurons
What is the minimum number of neurons in a reflex arc?
Inverse projection problem psychology
Functional classification of neurons
Autonomic motor neurons regulate visceral activities by
Mirror neurons wiki
Ganglia associated with efferent fibers
Image of neuron
Irritability of neurons
Decision support systems and intelligent systems
Nervous system and digestive system
Section 35-1 human body systems answer key
Comparison of endocrine and nervous system
Chapter 15 nervous system diseases and disorders
Exocrine glands are ductless
Difference between sympathetic and parasympathetic
Sympathetic and parasympathetic nervous system difference
Endocrine system and nervous system
Major division
Endocrine system vs nervous system
Somatic nervous system
Muscle and nervous tissue
Autonomic receptors
Principles of complex systems for systems engineering
Embedded systems vs cyber physical systems
Elegant systems
Chemical messengers of the nervous system
Platyhelminthes body segmentation
The nervous system is made up of
Neuron anatomy
Learning objectives of nervous system
Componentes componentes
What is stimuli in nervous system
Objectives of nervous system
Craniosacral region
Sympathetic nervous system def
Nerve plexus
Sns somatic nervous system
Brain scienstructable
Somatic motor neuron
Nematoda
Arthropods respiratory system
Chelicerates antennae
Are arthropods coelomates
Parasympathatic
Nervous system of coelenterata
Autonomic nervous system consists of
Doa nervous
L
Basic unit of nervous system
What is homeostasis
Histology of nervous system ppt
White matter
Sheep brain labeled
8 divisions of the nervous system
How to take care of your nervous system
Nervous system effector cells
Beta-blockers for overactive sympathetic nervous system
Somatic motor neuron
Spinal cord dorsal and ventral roots
Nervous system of silkworm
35-3 divisions of the nervous system
Women on the verge of a nervous breakdown summary
What is the basic building block of the nervous system
What does aunty em look like when she opens the door
Structure of nervous system graphic organizer
Medunerve
Classification of planaria
Phylum cnidaria polyp
Nervous
Divisions of the nervous system
Classify nervous tissue
Limb apraxia
Nervous system vocabulary
Disorders of the nervous system
Osteichthyes contoh
How the nervous system works