PCTH 400 Physicochemical Aspects of DrugReceptor Complexation Dr
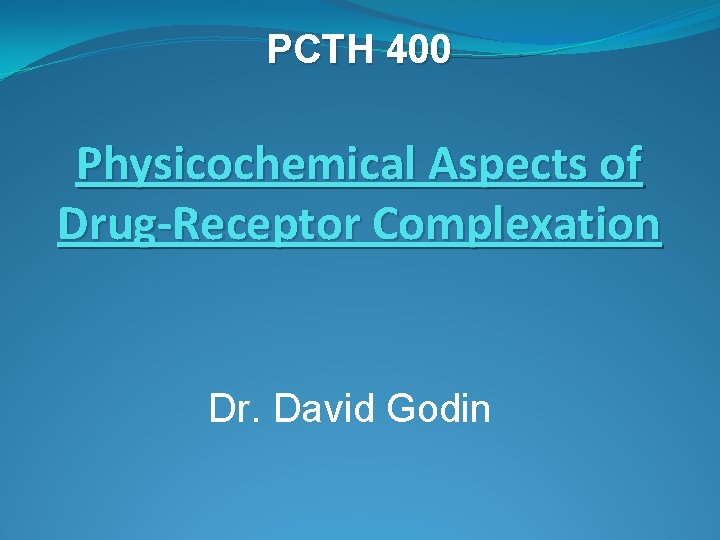
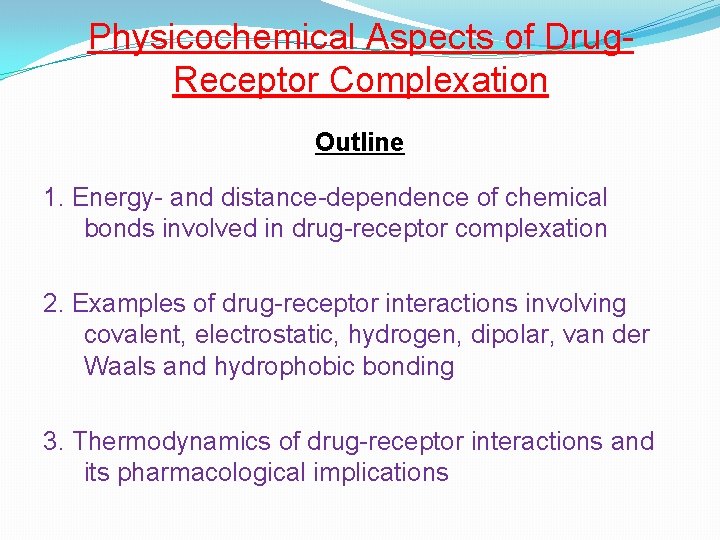
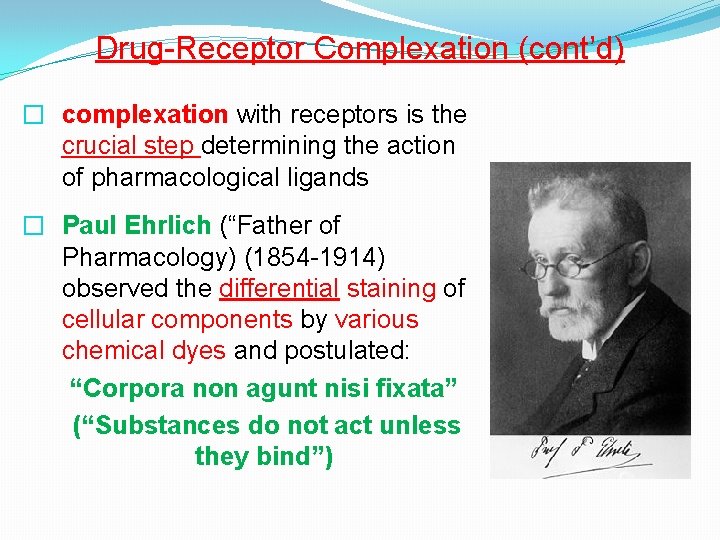
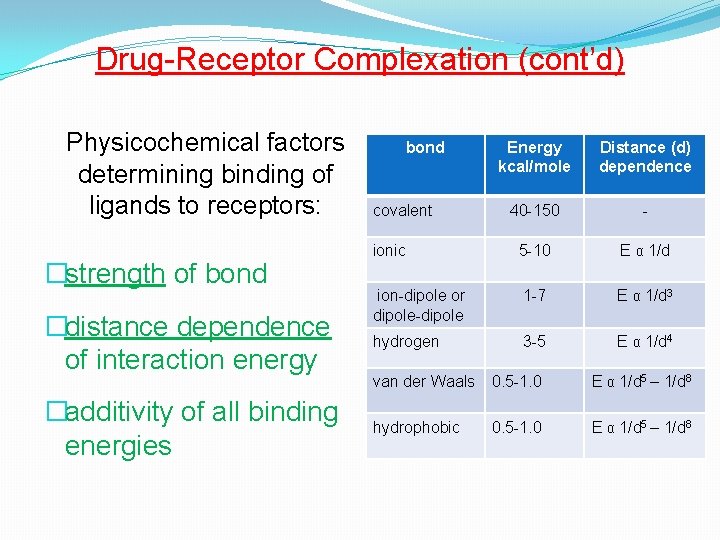
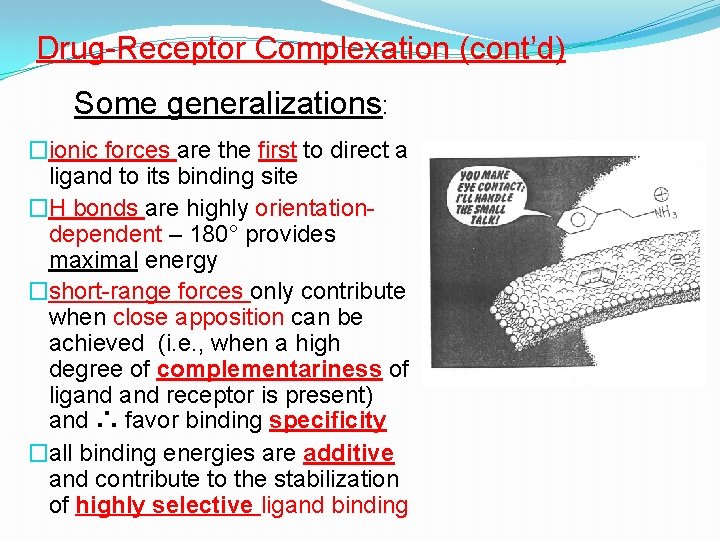
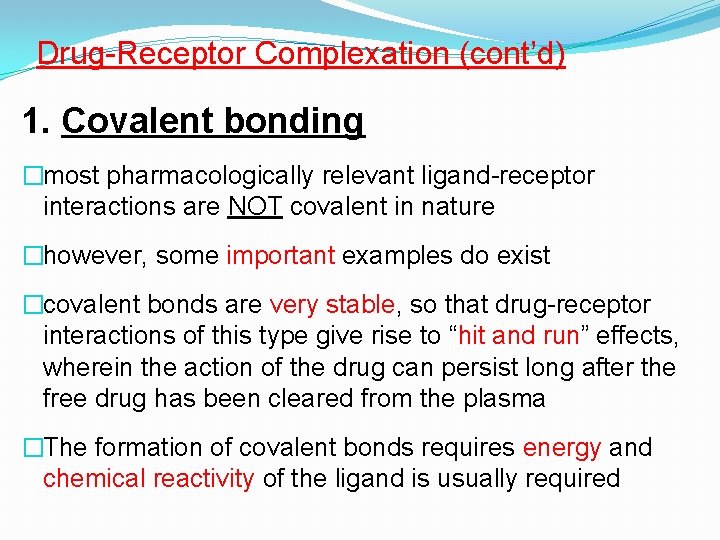
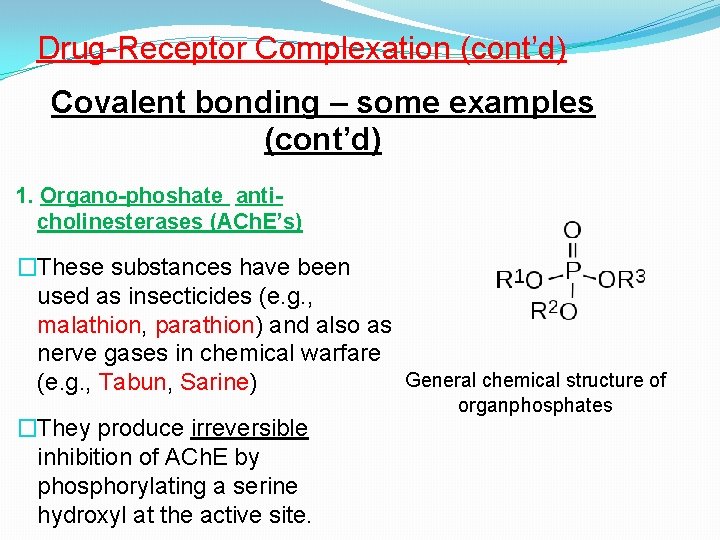
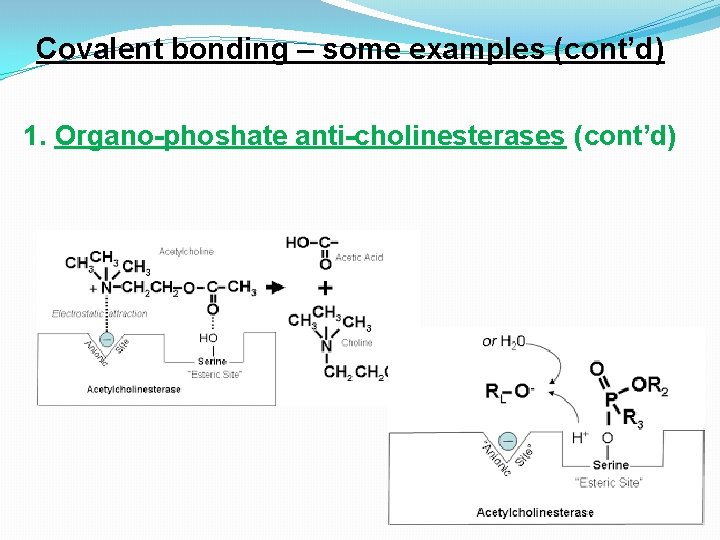
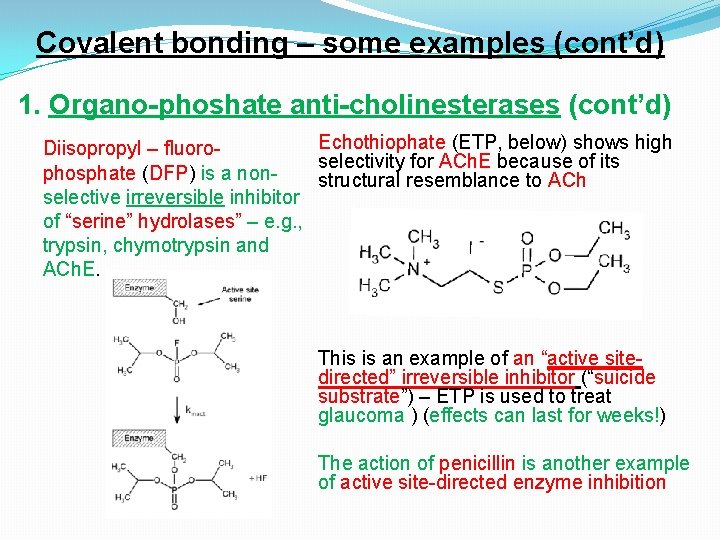
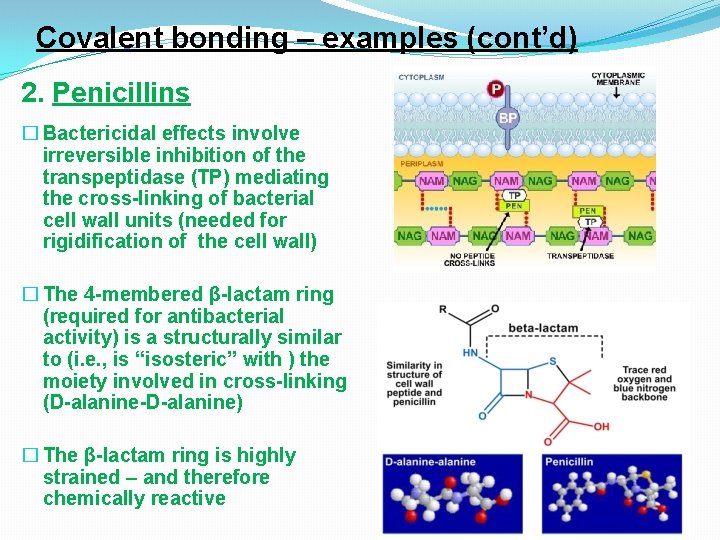
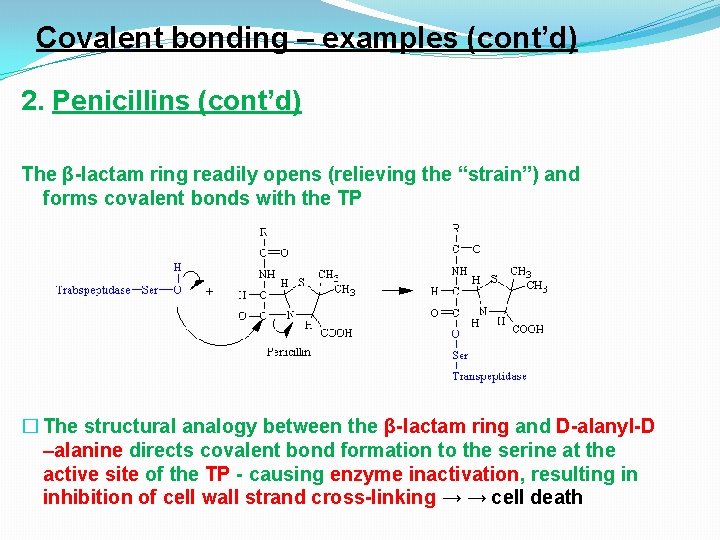
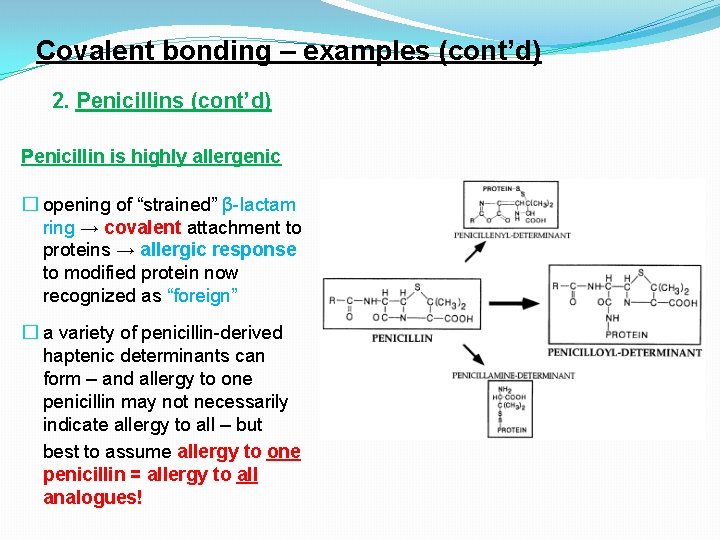
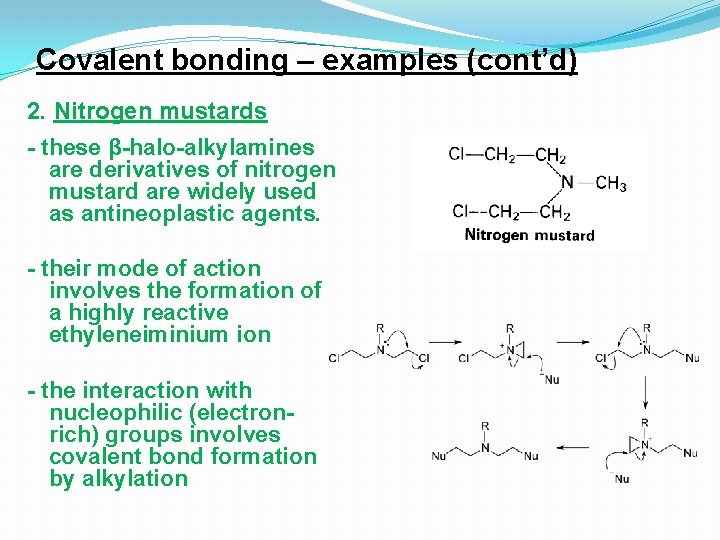
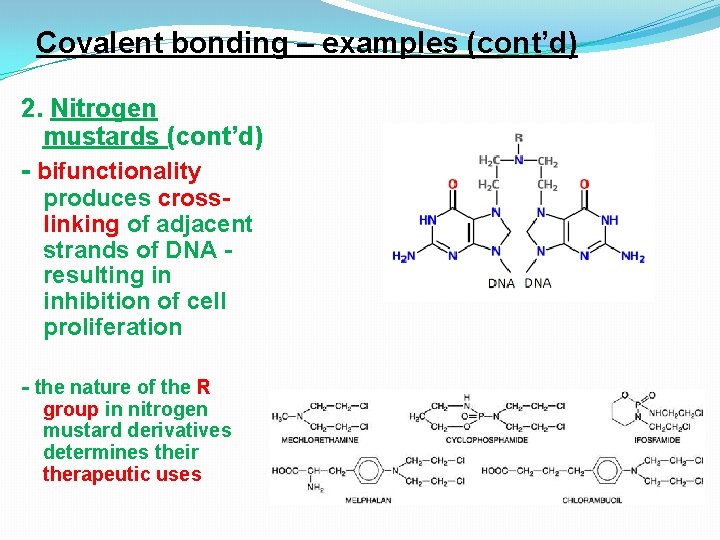
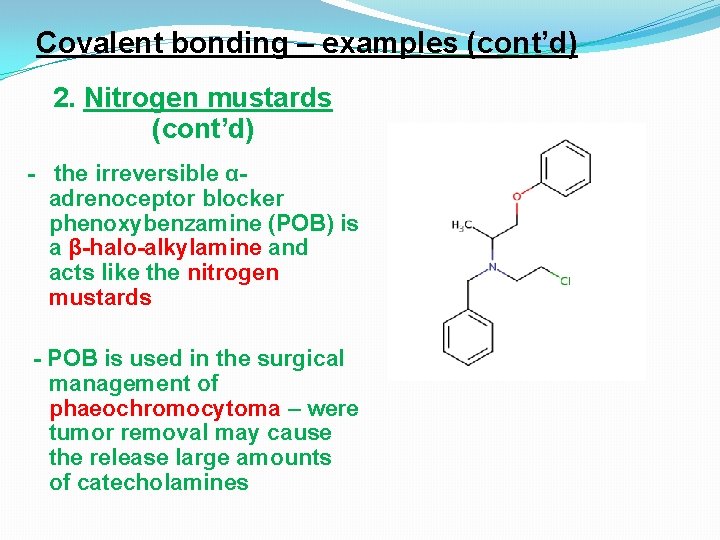
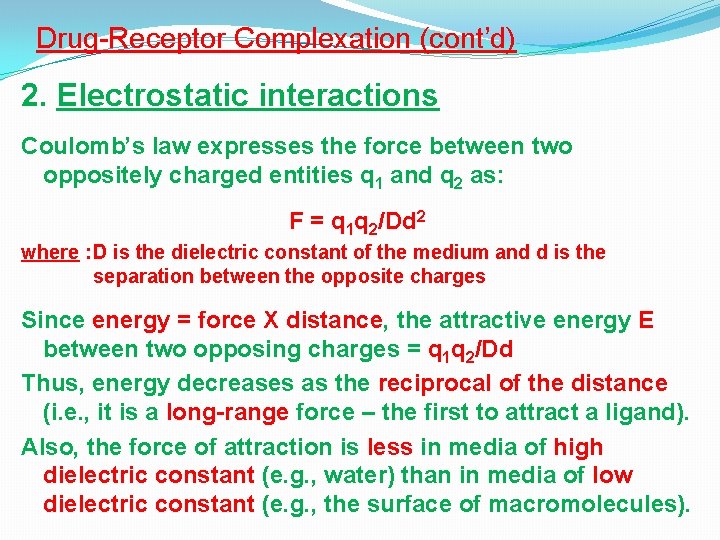
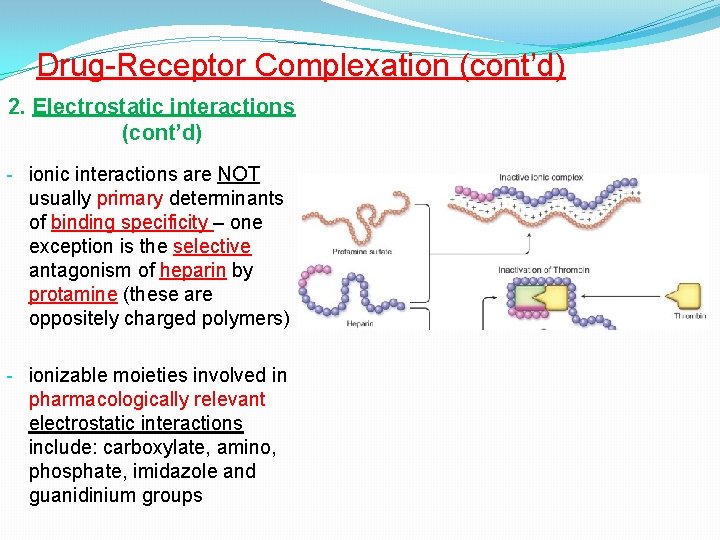
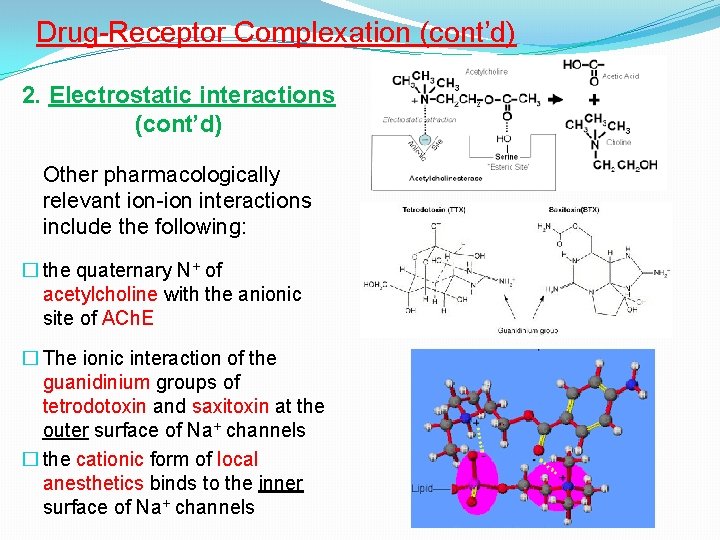
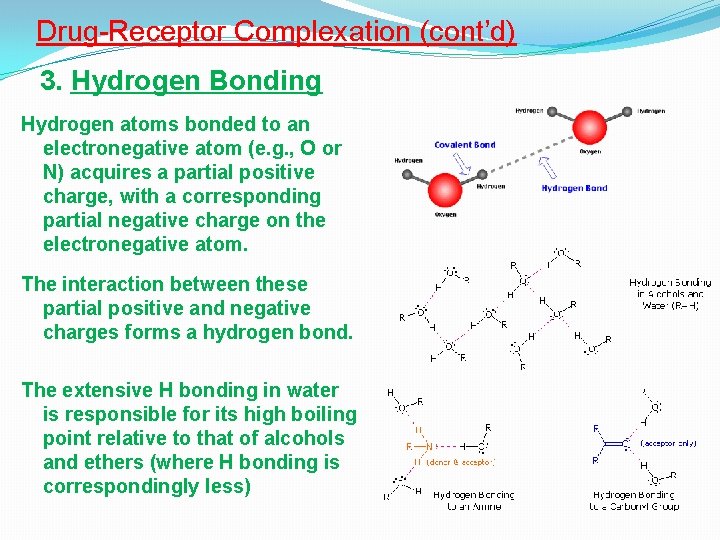
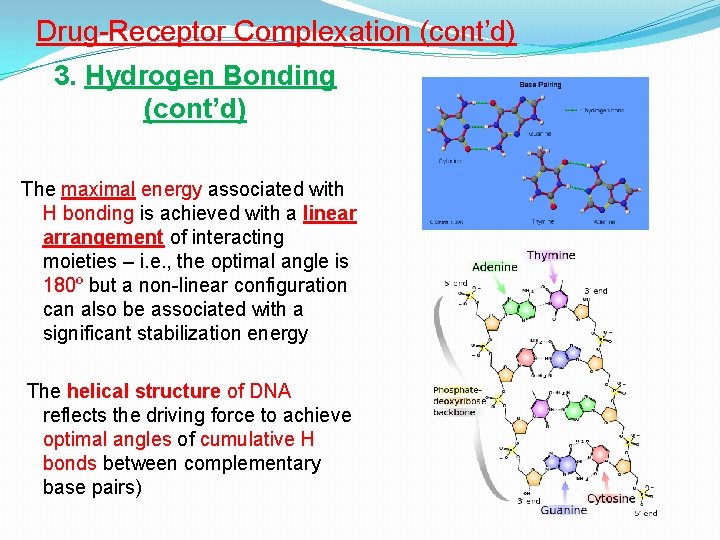
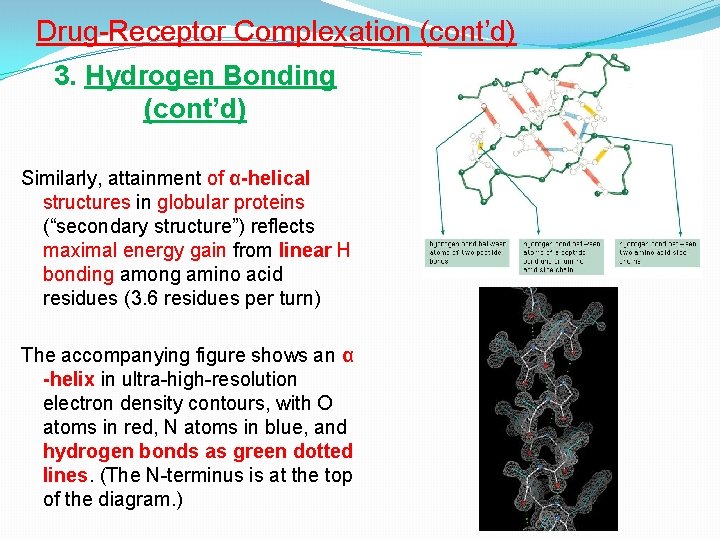
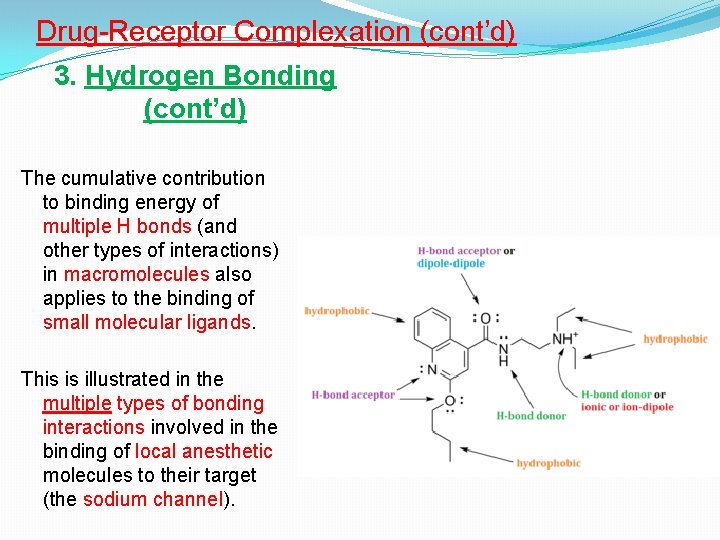
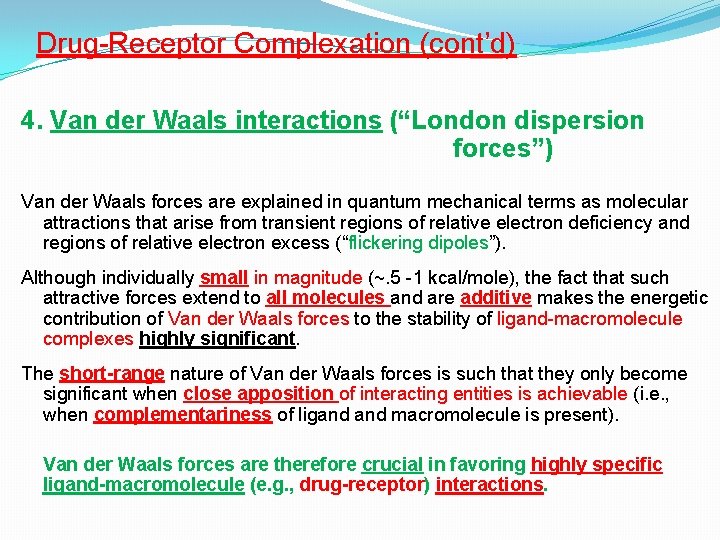
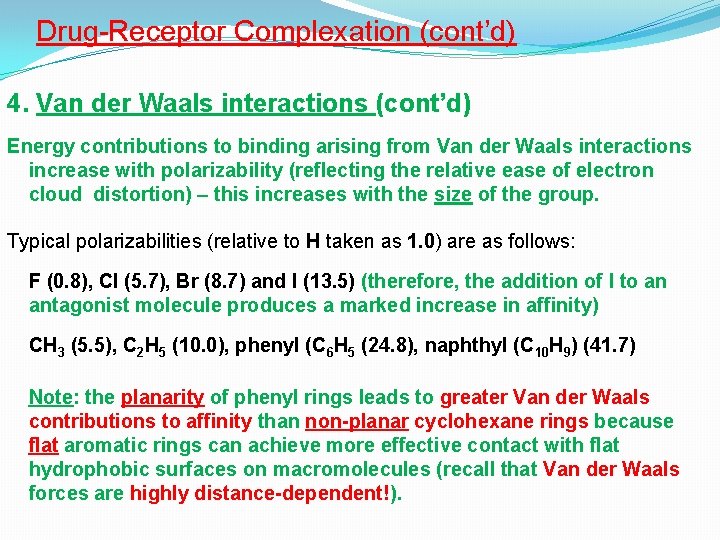
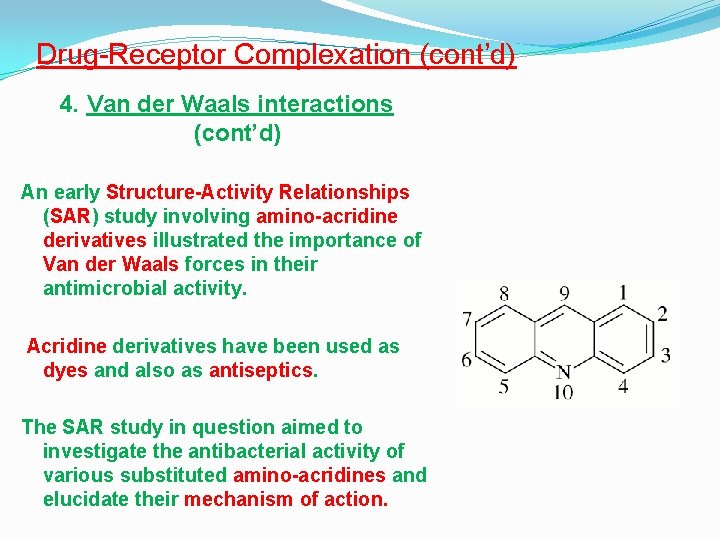
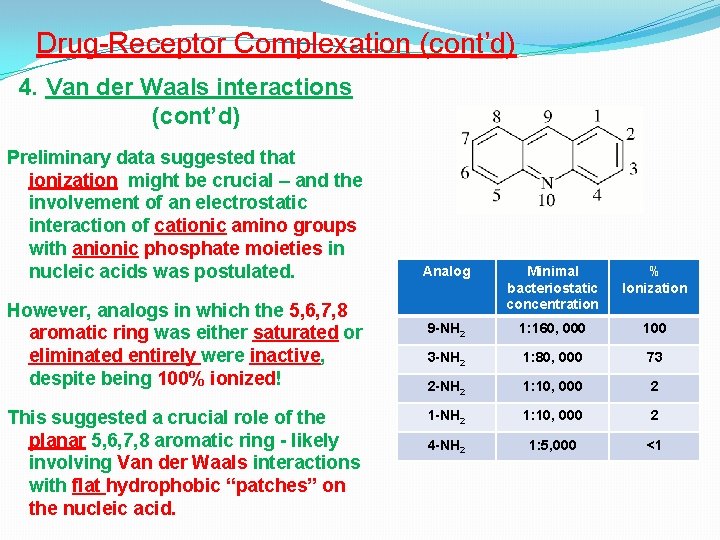
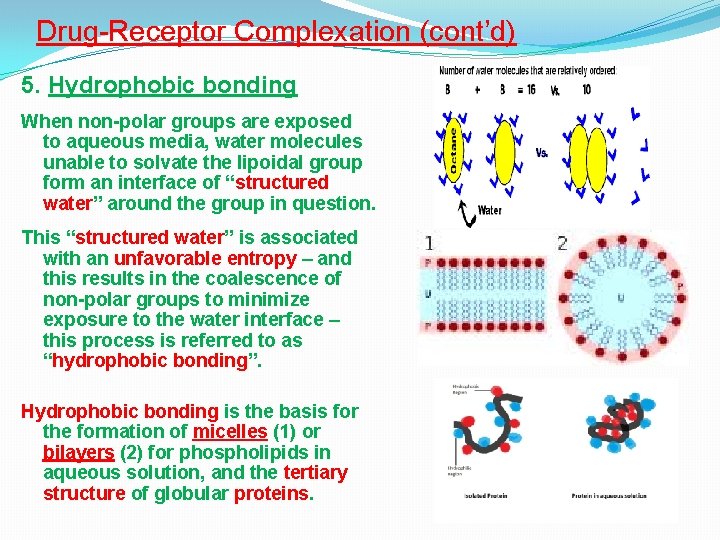
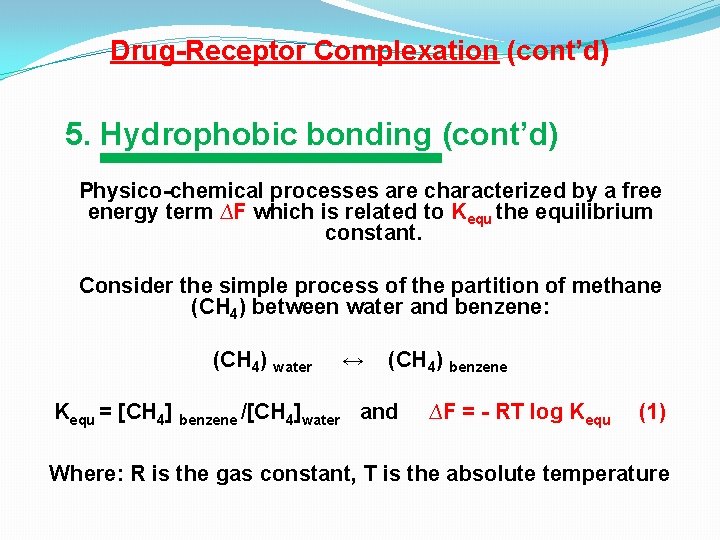
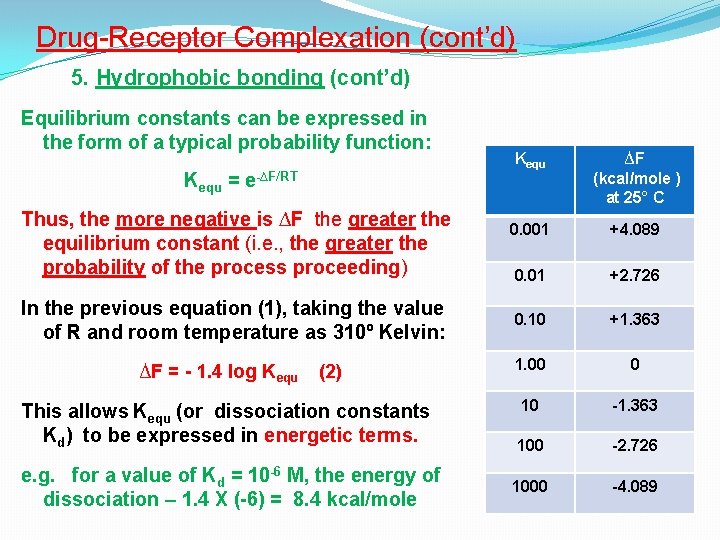
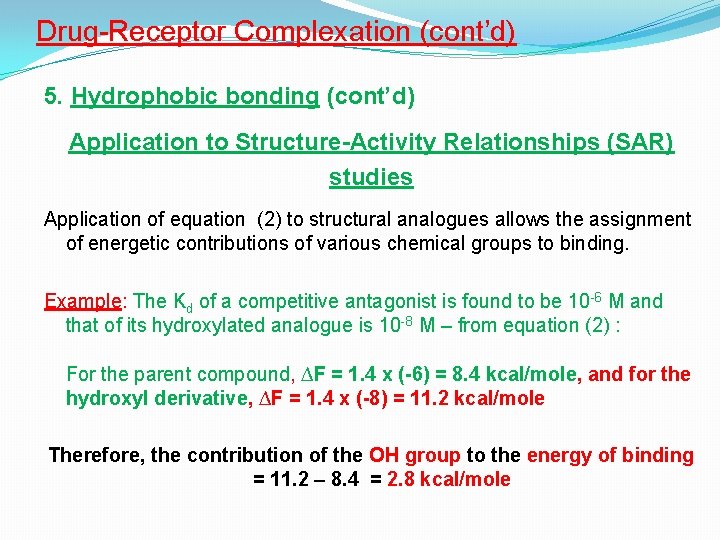
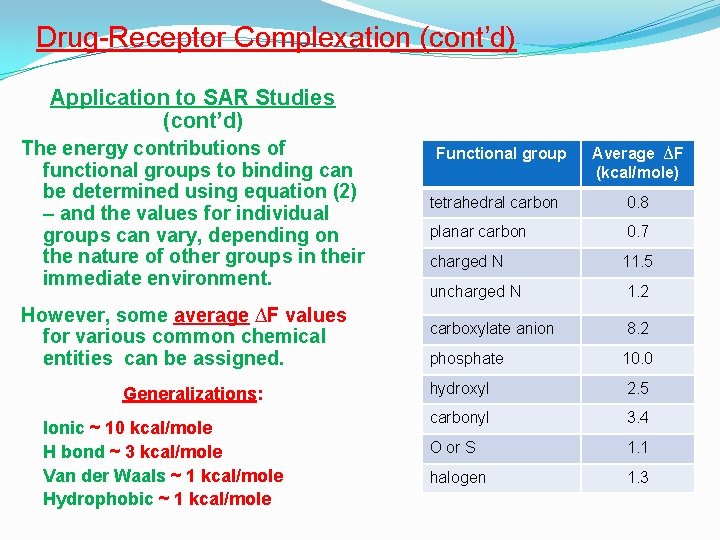
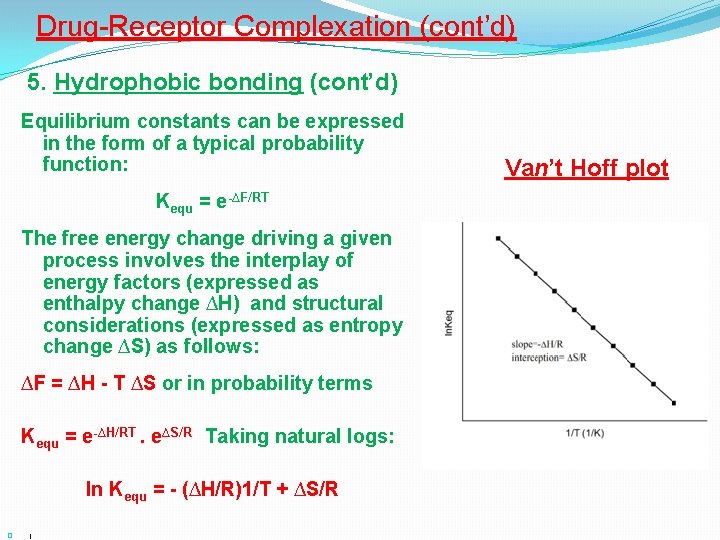
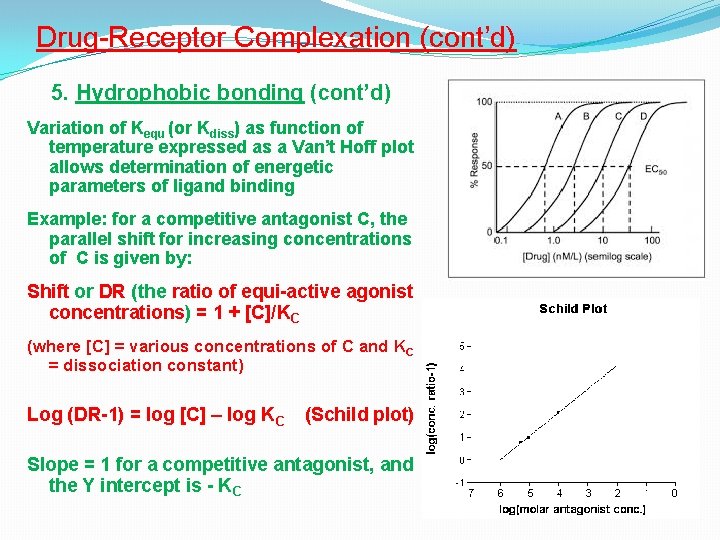
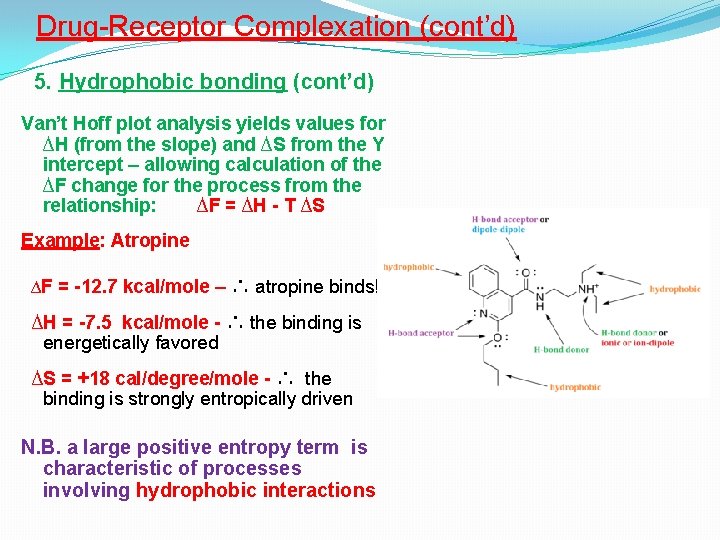
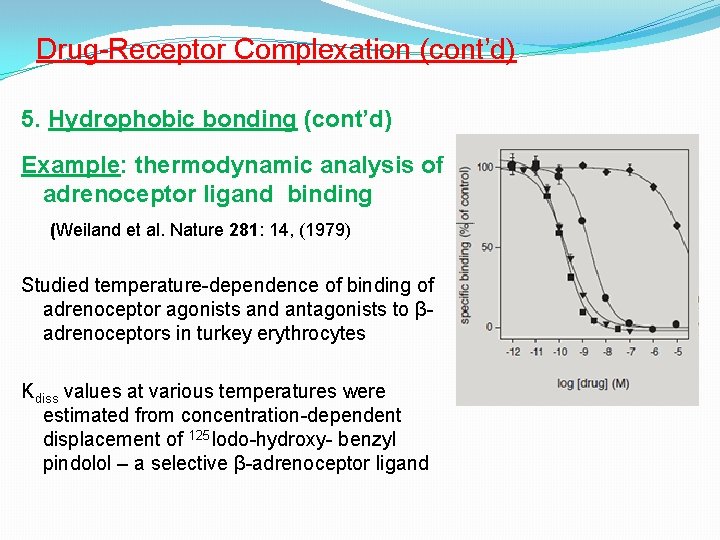
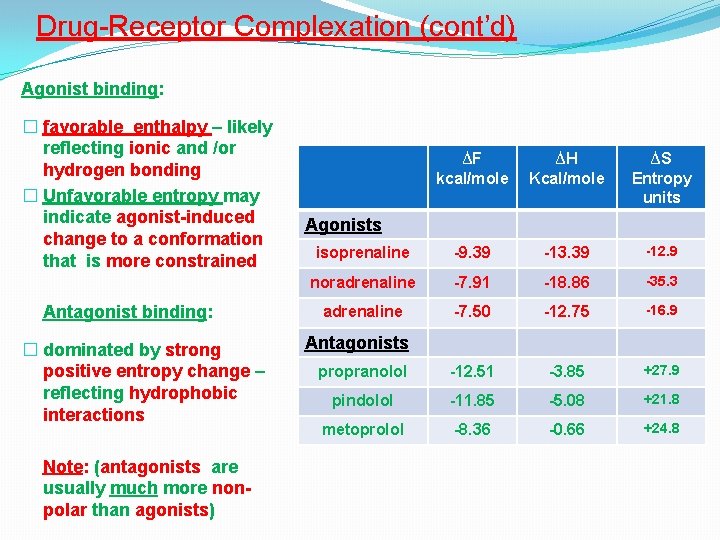
- Slides: 36
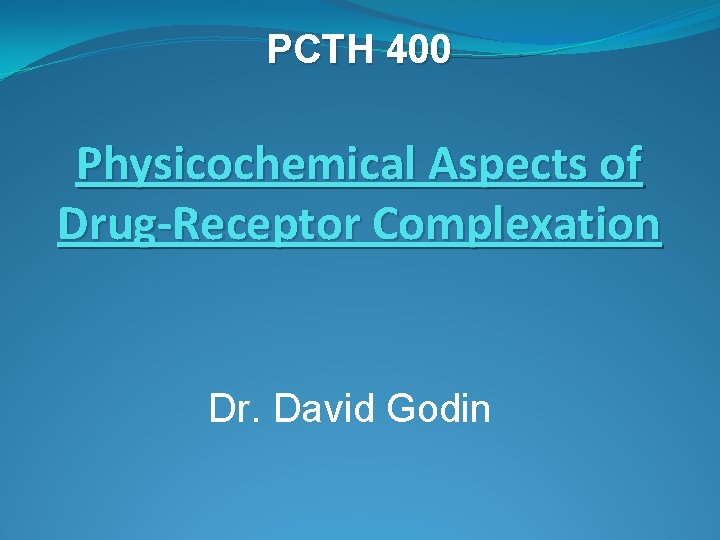
PCTH 400 Physicochemical Aspects of Drug-Receptor Complexation Dr. David Godin
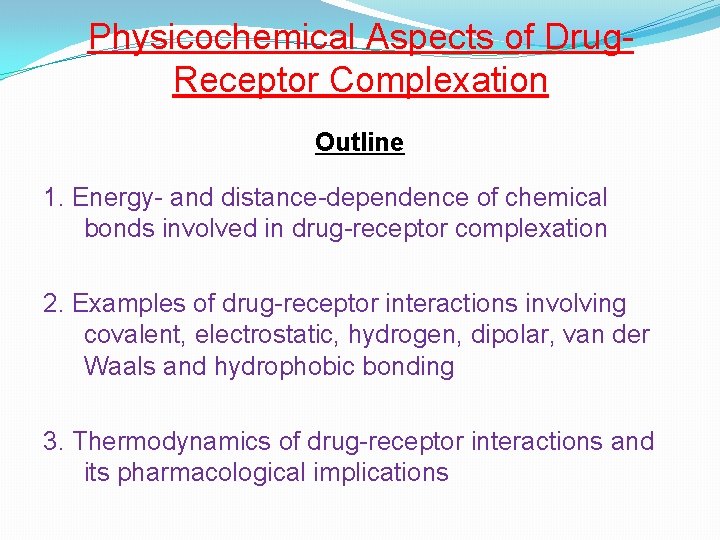
Physicochemical Aspects of Drug. Receptor Complexation Outline 1. Energy- and distance-dependence of chemical bonds involved in drug-receptor complexation 2. Examples of drug-receptor interactions involving covalent, electrostatic, hydrogen, dipolar, van der Waals and hydrophobic bonding 3. Thermodynamics of drug-receptor interactions and its pharmacological implications
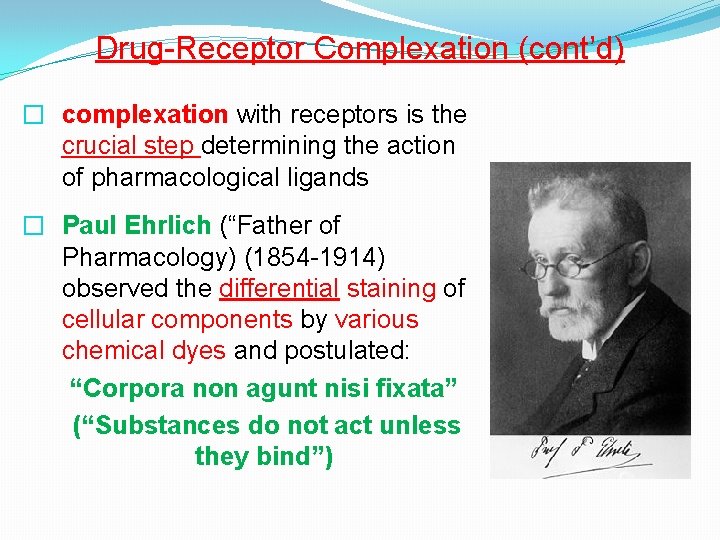
Drug-Receptor Complexation (cont’d) � complexation with receptors is the crucial step determining the action of pharmacological ligands � Paul Ehrlich (“Father of Pharmacology) (1854 -1914) observed the differential staining of cellular components by various chemical dyes and postulated: “Corpora non agunt nisi fixata” (“Substances do not act unless they bind”)
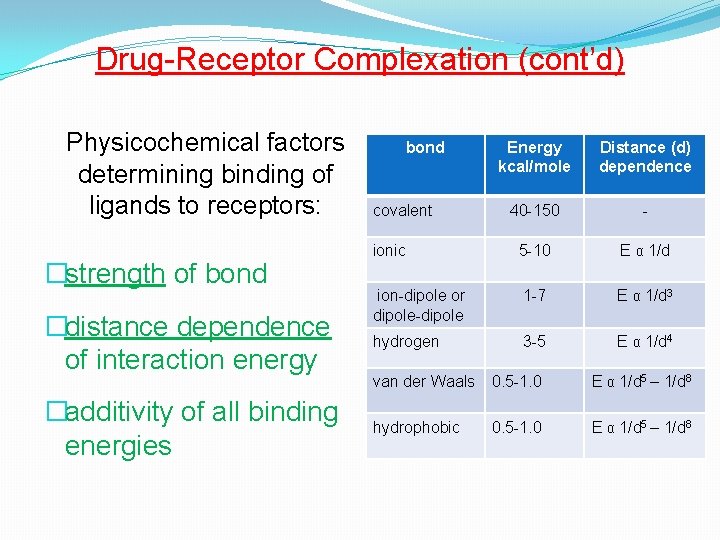
Drug-Receptor Complexation (cont’d) Physicochemical factors determining binding of ligands to receptors: �strength of bond �distance dependence of interaction energy �additivity of all binding energies bond Energy kcal/mole Distance (d) dependence 40 -150 - ionic 5 -10 E α 1/d ion-dipole or dipole-dipole 1 -7 E α 1/d 3 hydrogen 3 -5 E α 1/d 4 covalent van der Waals 0. 5 -1. 0 E α 1/d 5 – 1/d 8 hydrophobic 0. 5 -1. 0 E α 1/d 5 – 1/d 8
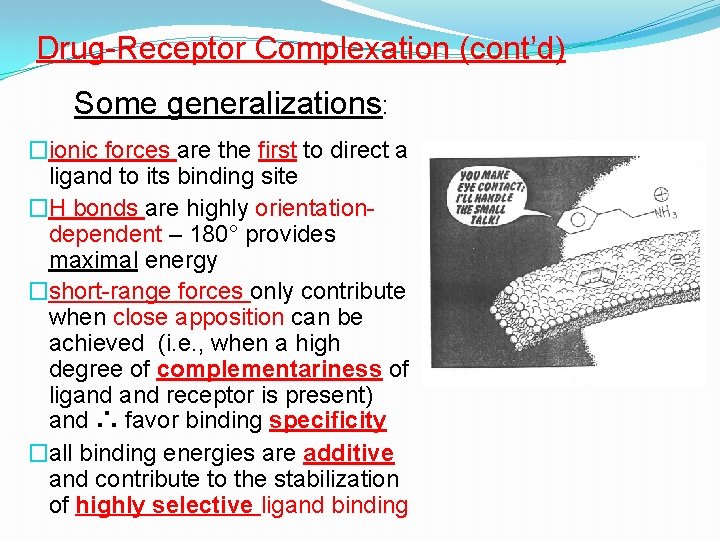
Drug-Receptor Complexation (cont’d) Some generalizations: �ionic forces are the first to direct a ligand to its binding site �H bonds are highly orientationdependent – 180° provides maximal energy �short-range forces only contribute when close apposition can be achieved (i. e. , when a high degree of complementariness of ligand receptor is present) and ∴ favor binding specificity �all binding energies are additive and contribute to the stabilization of highly selective ligand binding
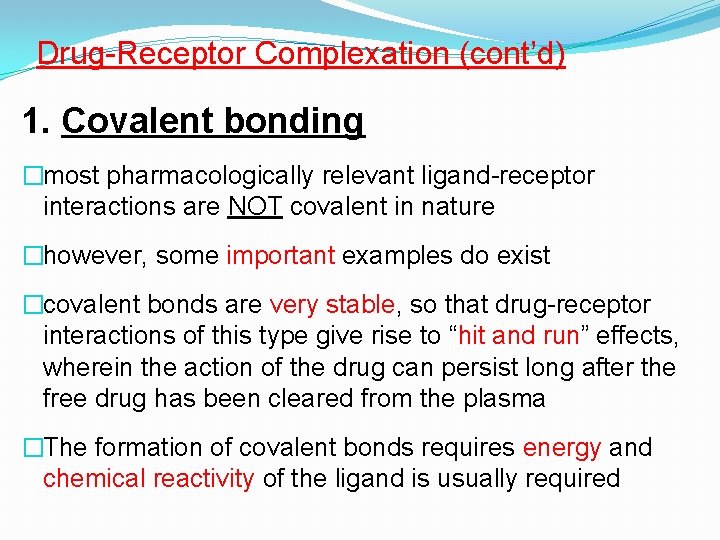
Drug-Receptor Complexation (cont’d) 1. Covalent bonding �most pharmacologically relevant ligand-receptor interactions are NOT covalent in nature �however, some important examples do exist �covalent bonds are very stable, so that drug-receptor interactions of this type give rise to “hit and run” effects, wherein the action of the drug can persist long after the free drug has been cleared from the plasma �The formation of covalent bonds requires energy and chemical reactivity of the ligand is usually required
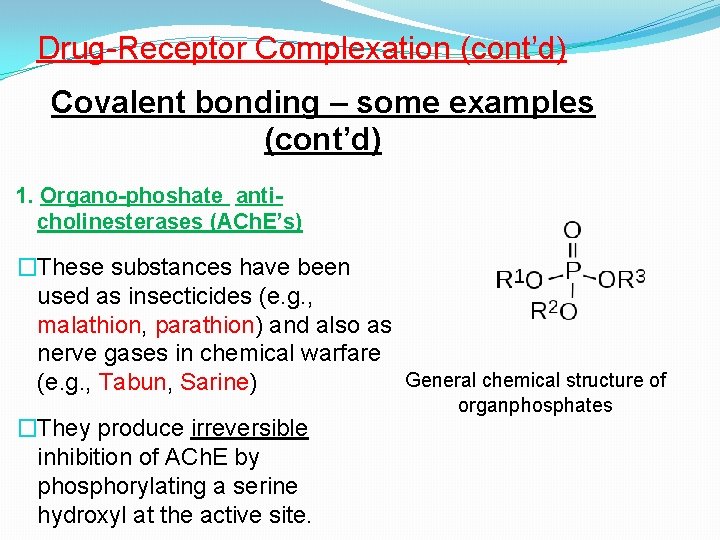
Drug-Receptor Complexation (cont’d) Covalent bonding – some examples (cont’d) 1. Organo-phoshate anticholinesterases (ACh. E’s) �These substances have been used as insecticides (e. g. , malathion, parathion) and also as nerve gases in chemical warfare General chemical structure of (e. g. , Tabun, Sarine) �They produce irreversible inhibition of ACh. E by phosphorylating a serine hydroxyl at the active site. organphosphates
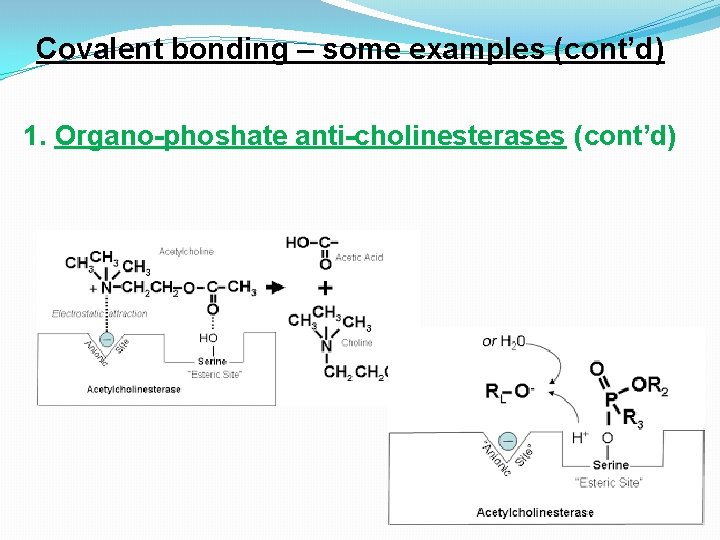
Covalent bonding – some examples (cont’d) 1. Organo-phoshate anti-cholinesterases (cont’d)
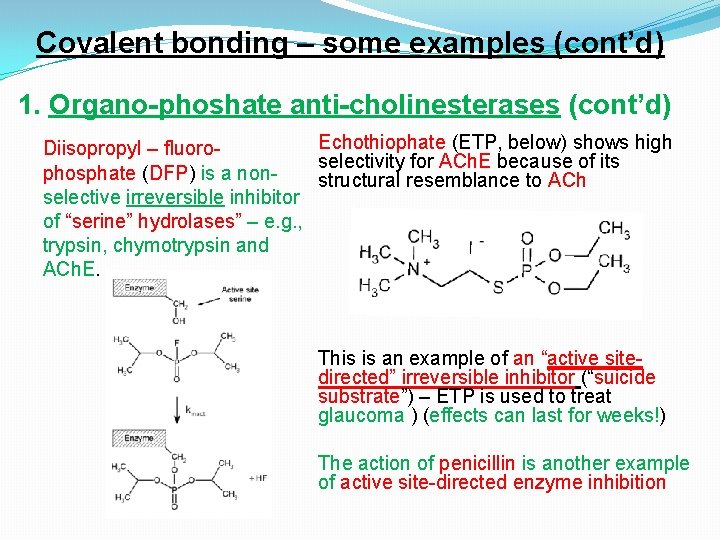
Covalent bonding – some examples (cont’d) 1. Organo-phoshate anti-cholinesterases (cont’d) Echothiophate (ETP, below) shows high Diisopropyl – fluoroselectivity for ACh. E because of its phosphate (DFP) is a nonstructural resemblance to ACh selective irreversible inhibitor of “serine” hydrolases” – e. g. , trypsin, chymotrypsin and ACh. E. This is an example of an “active sitedirected” irreversible inhibitor (“suicide substrate”) – ETP is used to treat glaucoma ) (effects can last for weeks!) The action of penicillin is another example of active site-directed enzyme inhibition
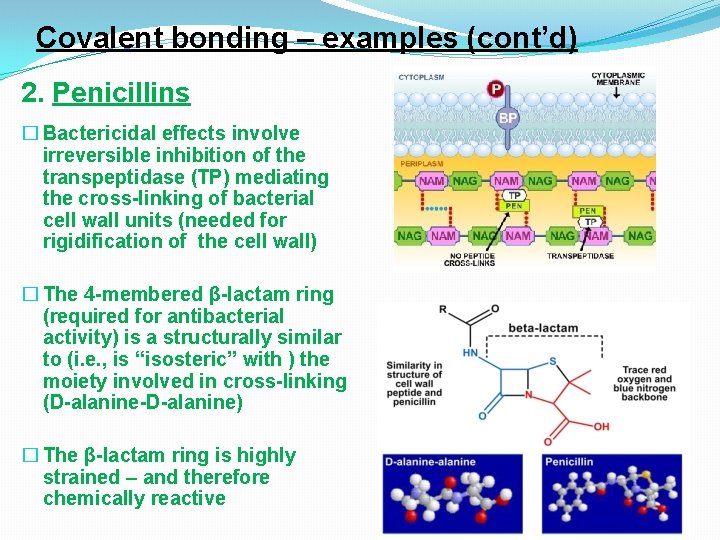
Covalent bonding – examples (cont’d) 2. Penicillins � Bactericidal effects involve irreversible inhibition of the transpeptidase (TP) mediating the cross-linking of bacterial cell wall units (needed for rigidification of the cell wall) � The 4 -membered β-lactam ring (required for antibacterial activity) is a structurally similar to (i. e. , is “isosteric” with ) the moiety involved in cross-linking (D-alanine-D-alanine) � The β-lactam ring is highly strained – and therefore chemically reactive
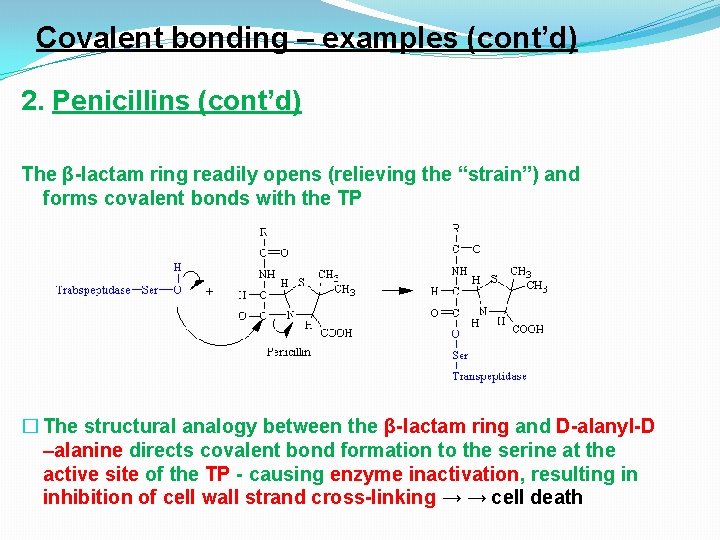
Covalent bonding – examples (cont’d) 2. Penicillins (cont’d) The β-lactam ring readily opens (relieving the “strain”) and forms covalent bonds with the TP � The structural analogy between the β-lactam ring and D-alanyl-D –alanine directs covalent bond formation to the serine at the active site of the TP - causing enzyme inactivation, resulting in inhibition of cell wall strand cross-linking → → cell death
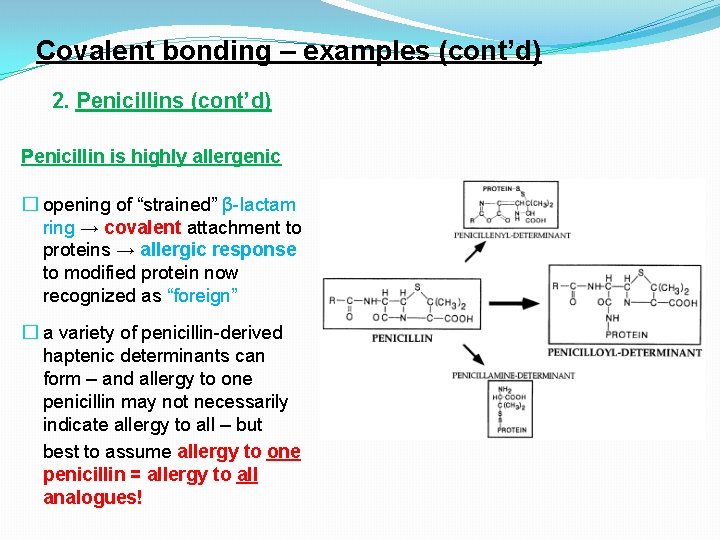
Covalent bonding – examples (cont’d) 2. Penicillins (cont’d) Penicillin is highly allergenic � opening of “strained” β-lactam ring → covalent attachment to proteins → allergic response to modified protein now recognized as “foreign” � a variety of penicillin-derived haptenic determinants can form – and allergy to one penicillin may not necessarily indicate allergy to all – but best to assume allergy to one penicillin = allergy to all analogues!
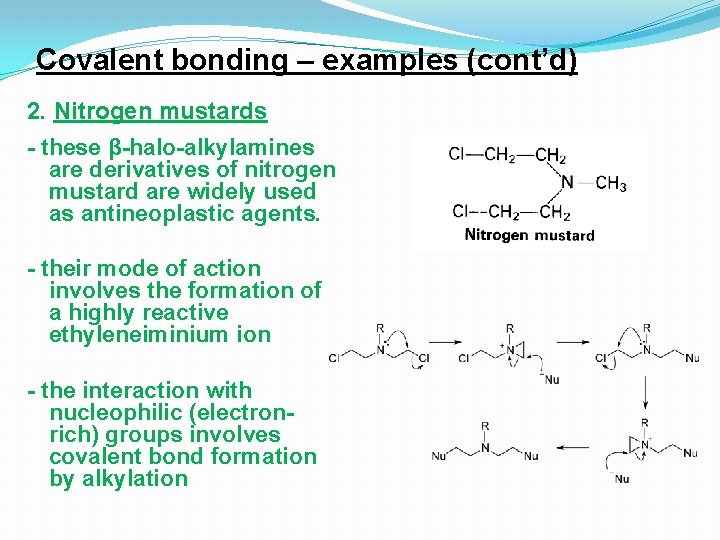
Covalent bonding – examples (cont’d) 2. Nitrogen mustards - these β-halo-alkylamines are derivatives of nitrogen mustard are widely used as antineoplastic agents. - their mode of action involves the formation of a highly reactive ethyleneiminium ion - the interaction with nucleophilic (electronrich) groups involves covalent bond formation by alkylation
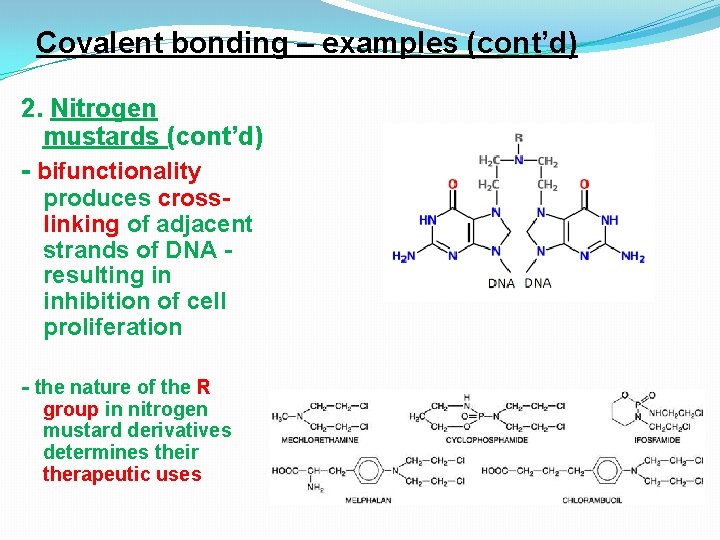
Covalent bonding – examples (cont’d) 2. Nitrogen mustards (cont’d) - bifunctionality produces crosslinking of adjacent strands of DNA resulting in inhibition of cell proliferation - the nature of the R group in nitrogen mustard derivatives determines their therapeutic uses
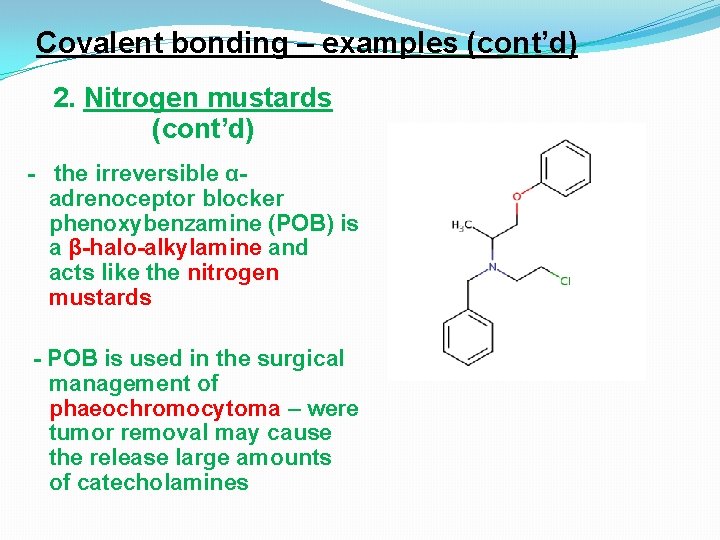
Covalent bonding – examples (cont’d) 2. Nitrogen mustards (cont’d) - the irreversible αadrenoceptor blocker phenoxybenzamine (POB) is a β-halo-alkylamine and acts like the nitrogen mustards - POB is used in the surgical management of phaeochromocytoma – were tumor removal may cause the release large amounts of catecholamines
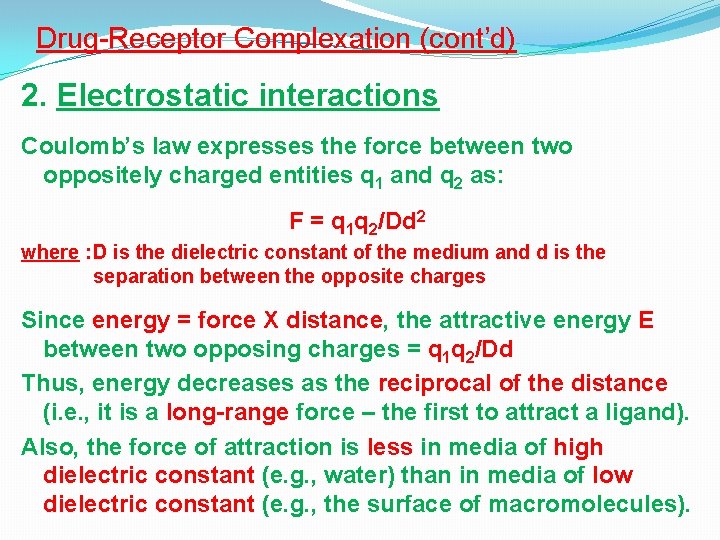
Drug-Receptor Complexation (cont’d) 2. Electrostatic interactions Coulomb’s law expresses the force between two oppositely charged entities q 1 and q 2 as: F = q 1 q 2/Dd 2 where : D is the dielectric constant of the medium and d is the separation between the opposite charges Since energy = force X distance, the attractive energy E between two opposing charges = q 1 q 2/Dd Thus, energy decreases as the reciprocal of the distance (i. e. , it is a long-range force – the first to attract a ligand). Also, the force of attraction is less in media of high dielectric constant (e. g. , water) than in media of low dielectric constant (e. g. , the surface of macromolecules).
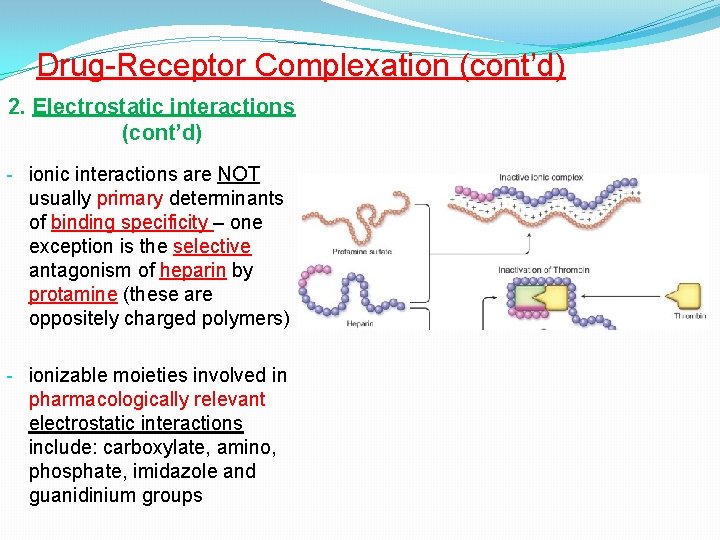
Drug-Receptor Complexation (cont’d) 2. Electrostatic interactions (cont’d) - ionic interactions are NOT usually primary determinants of binding specificity – one exception is the selective antagonism of heparin by protamine (these are oppositely charged polymers) - ionizable moieties involved in pharmacologically relevant electrostatic interactions include: carboxylate, amino, phosphate, imidazole and guanidinium groups
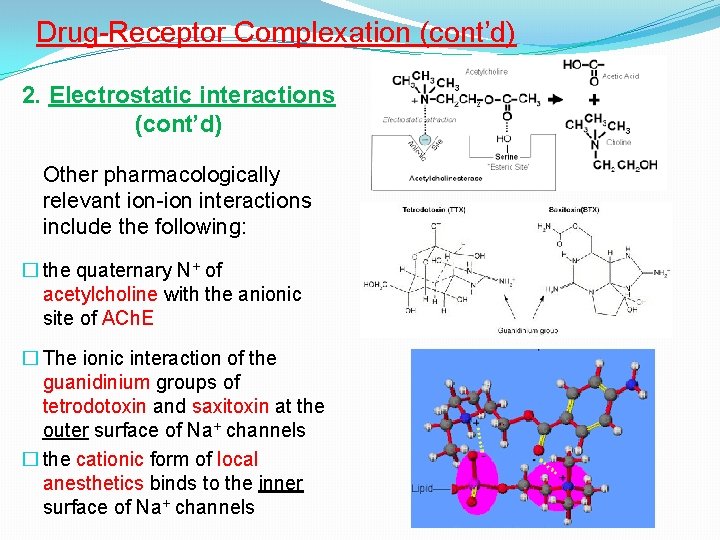
Drug-Receptor Complexation (cont’d) 2. Electrostatic interactions (cont’d) Other pharmacologically relevant ion-ion interactions include the following: � the quaternary N+ of acetylcholine with the anionic site of ACh. E � The ionic interaction of the guanidinium groups of tetrodotoxin and saxitoxin at the outer surface of Na+ channels � the cationic form of local anesthetics binds to the inner surface of Na+ channels
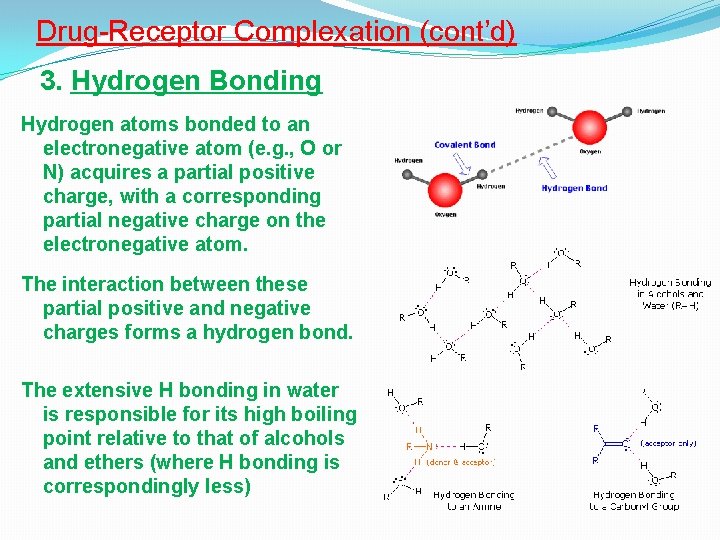
Drug-Receptor Complexation (cont’d) 3. Hydrogen Bonding Hydrogen atoms bonded to an electronegative atom (e. g. , O or N) acquires a partial positive charge, with a corresponding partial negative charge on the electronegative atom. The interaction between these partial positive and negative charges forms a hydrogen bond. The extensive H bonding in water is responsible for its high boiling point relative to that of alcohols and ethers (where H bonding is correspondingly less)
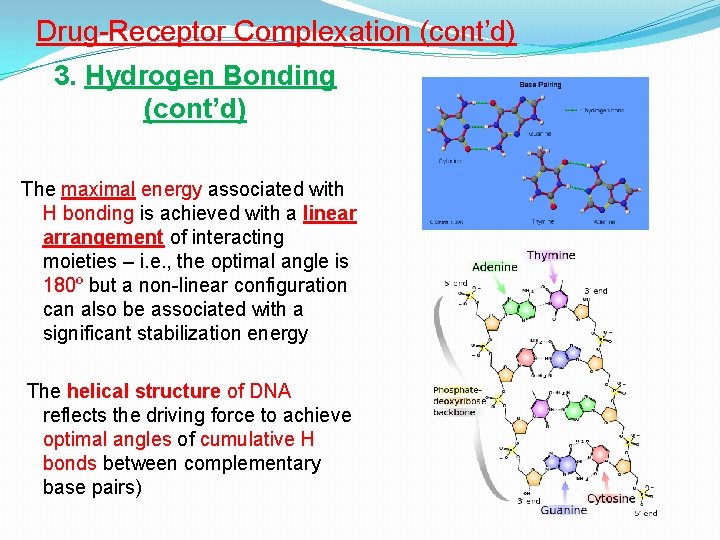
Drug-Receptor Complexation (cont’d) 3. Hydrogen Bonding (cont’d) The maximal energy associated with H bonding is achieved with a linear arrangement of interacting moieties – i. e. , the optimal angle is 180º but a non-linear configuration can also be associated with a significant stabilization energy The helical structure of DNA reflects the driving force to achieve optimal angles of cumulative H bonds between complementary base pairs)
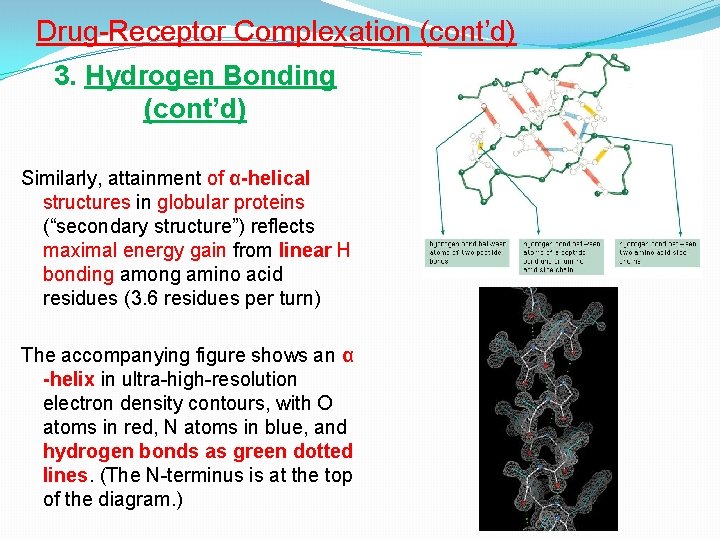
Drug-Receptor Complexation (cont’d) 3. Hydrogen Bonding (cont’d) Similarly, attainment of α-helical structures in globular proteins (“secondary structure”) reflects maximal energy gain from linear H bonding among amino acid residues (3. 6 residues per turn) The accompanying figure shows an α -helix in ultra-high-resolution electron density contours, with O atoms in red, N atoms in blue, and hydrogen bonds as green dotted lines. (The N-terminus is at the top of the diagram. )
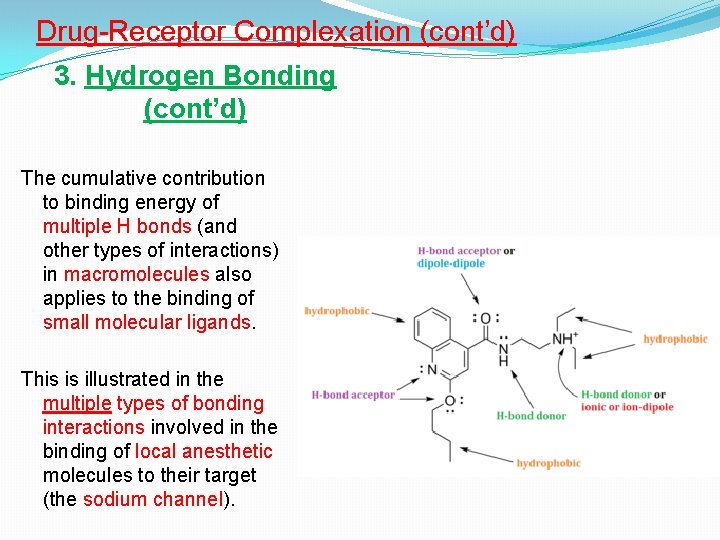
Drug-Receptor Complexation (cont’d) 3. Hydrogen Bonding (cont’d) The cumulative contribution to binding energy of multiple H bonds (and other types of interactions) in macromolecules also applies to the binding of small molecular ligands. This is illustrated in the multiple types of bonding interactions involved in the binding of local anesthetic molecules to their target (the sodium channel).
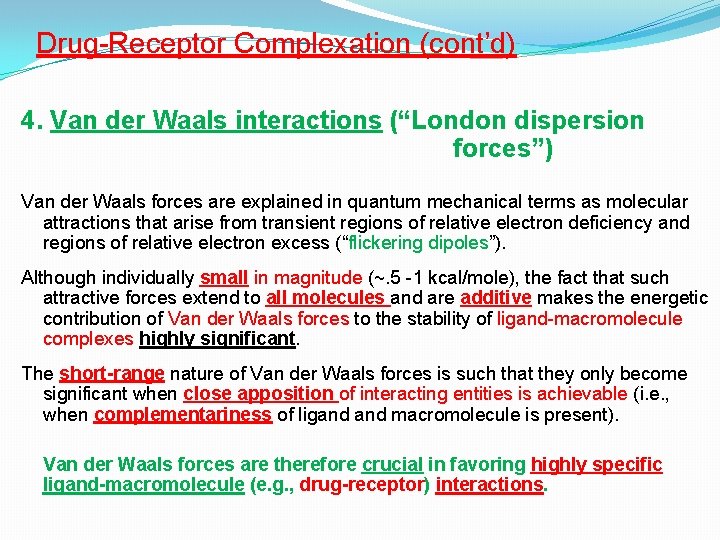
Drug-Receptor Complexation (cont’d) 4. Van der Waals interactions (“London dispersion forces”) Van der Waals forces are explained in quantum mechanical terms as molecular attractions that arise from transient regions of relative electron deficiency and regions of relative electron excess (“flickering dipoles”). Although individually small in magnitude (~. 5 -1 kcal/mole), the fact that such attractive forces extend to all molecules and are additive makes the energetic contribution of Van der Waals forces to the stability of ligand-macromolecule complexes highly significant. The short-range nature of Van der Waals forces is such that they only become significant when close apposition of interacting entities is achievable (i. e. , when complementariness of ligand macromolecule is present). Van der Waals forces are therefore crucial in favoring highly specific ligand-macromolecule (e. g. , drug-receptor) interactions.
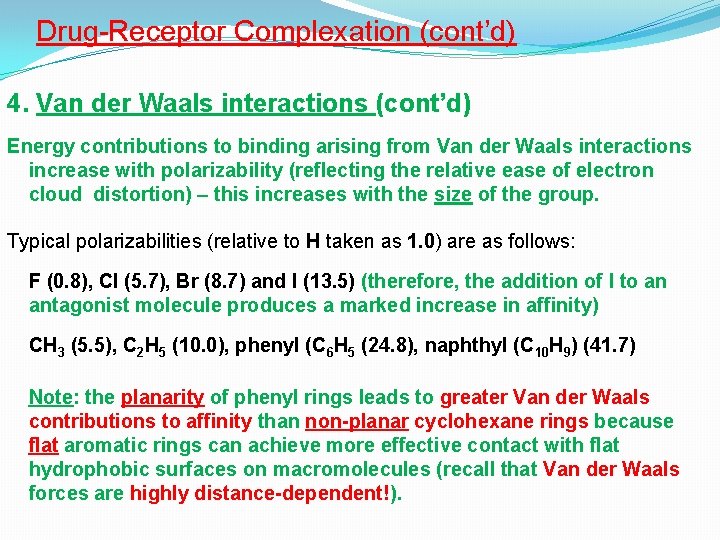
Drug-Receptor Complexation (cont’d) 4. Van der Waals interactions (cont’d) Energy contributions to binding arising from Van der Waals interactions increase with polarizability (reflecting the relative ease of electron cloud distortion) – this increases with the size of the group. Typical polarizabilities (relative to H taken as 1. 0) are as follows: F (0. 8), Cl (5. 7), Br (8. 7) and I (13. 5) (therefore, the addition of I to an antagonist molecule produces a marked increase in affinity) CH 3 (5. 5), C 2 H 5 (10. 0), phenyl (C 6 H 5 (24. 8), naphthyl (C 10 H 9) (41. 7) Note: the planarity of phenyl rings leads to greater Van der Waals contributions to affinity than non-planar cyclohexane rings because flat aromatic rings can achieve more effective contact with flat hydrophobic surfaces on macromolecules (recall that Van der Waals forces are highly distance-dependent!).
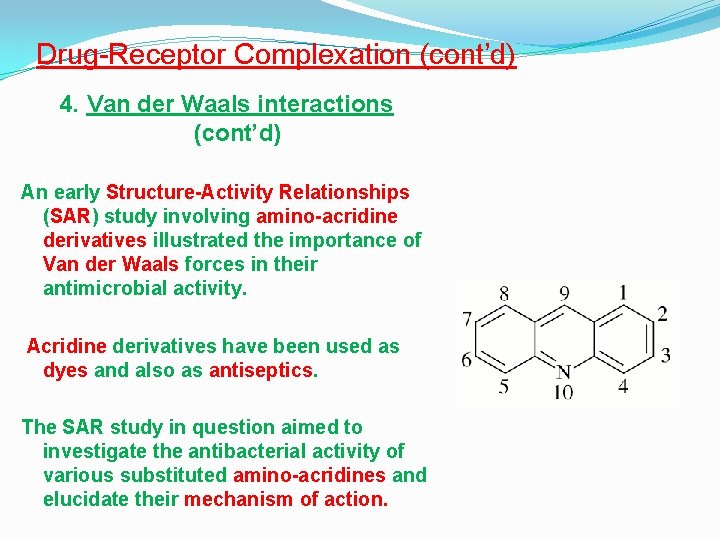
Drug-Receptor Complexation (cont’d) 4. Van der Waals interactions (cont’d) An early Structure-Activity Relationships (SAR) study involving amino-acridine derivatives illustrated the importance of Van der Waals forces in their antimicrobial activity. Acridine derivatives have been used as dyes and also as antiseptics. The SAR study in question aimed to investigate the antibacterial activity of various substituted amino-acridines and elucidate their mechanism of action.
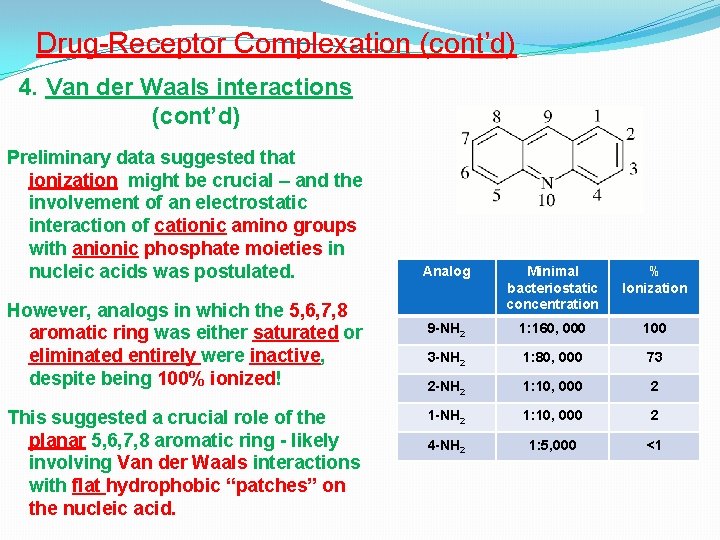
Drug-Receptor Complexation (cont’d) 4. Van der Waals interactions (cont’d) Preliminary data suggested that ionization might be crucial – and the involvement of an electrostatic interaction of cationic amino groups with anionic phosphate moieties in nucleic acids was postulated. However, analogs in which the 5, 6, 7, 8 aromatic ring was either saturated or eliminated entirely were inactive, despite being 100% ionized! This suggested a crucial role of the planar 5, 6, 7, 8 aromatic ring - likely involving Van der Waals interactions with flat hydrophobic “patches” on the nucleic acid. Analog Minimal bacteriostatic concentration % Ionization 9 -NH 2 1: 160, 000 100 3 -NH 2 1: 80, 000 73 2 -NH 2 1: 10, 000 2 1 -NH 2 1: 10, 000 2 4 -NH 2 1: 5, 000 <1
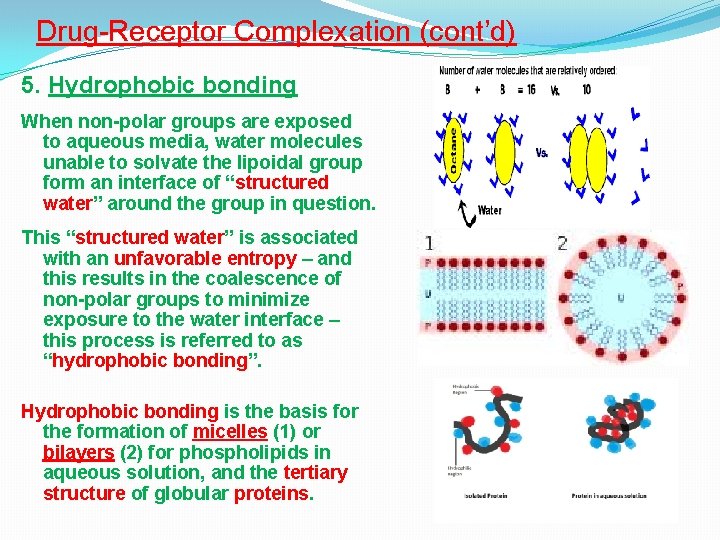
Drug-Receptor Complexation (cont’d) 5. Hydrophobic bonding When non-polar groups are exposed to aqueous media, water molecules unable to solvate the lipoidal group form an interface of “structured water” around the group in question. This “structured water” is associated with an unfavorable entropy – and this results in the coalescence of non-polar groups to minimize exposure to the water interface – this process is referred to as “hydrophobic bonding”. Hydrophobic bonding is the basis for the formation of micelles (1) or bilayers (2) for phospholipids in aqueous solution, and the tertiary structure of globular proteins.
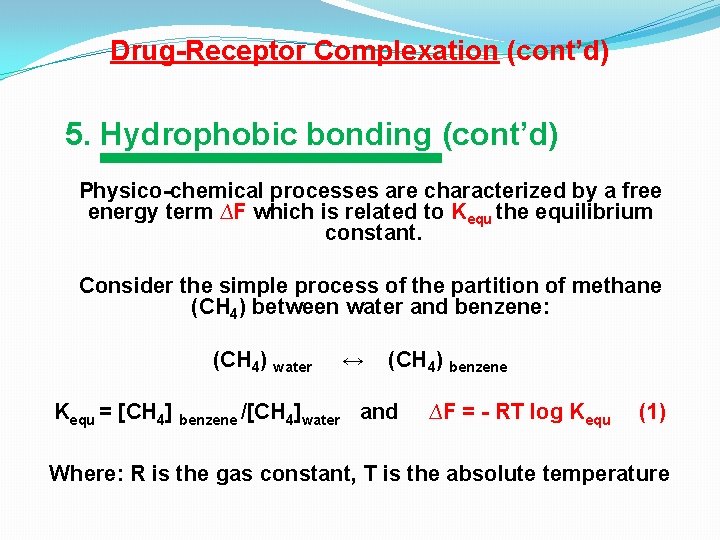
Drug-Receptor Complexation (cont’d) 5. Hydrophobic bonding (cont’d) Physico-chemical processes are characterized by a free energy term ∆F which is related to Kequ the equilibrium constant. Consider the simple process of the partition of methane (CH 4) between water and benzene: (CH 4) water ↔ (CH 4) benzene Kequ = [CH 4] benzene /[CH 4]water and ∆F = - RT log Kequ (1) Where: R is the gas constant, T is the absolute temperature
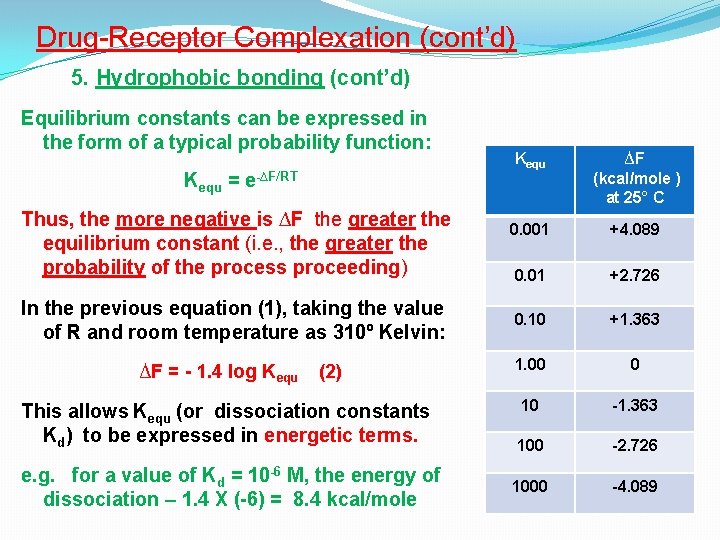
Drug-Receptor Complexation (cont’d) 5. Hydrophobic bonding (cont’d) Equilibrium constants can be expressed in the form of a typical probability function: Kequ = e-∆F/RT Thus, the more negative is ∆F the greater the equilibrium constant (i. e. , the greater the probability of the process proceeding) In the previous equation (1), taking the value of R and room temperature as 310º Kelvin: ∆F = - 1. 4 log Kequ (2) This allows Kequ (or dissociation constants Kd) to be expressed in energetic terms. e. g. for a value of Kd = 10 -6 M, the energy of dissociation – 1. 4 X (-6) = 8. 4 kcal/mole Kequ ∆F (kcal/mole ) at 25° C 0. 001 +4. 089 0. 01 +2. 726 0. 10 +1. 363 1. 00 0 10 -1. 363 100 -2. 726 1000 -4. 089
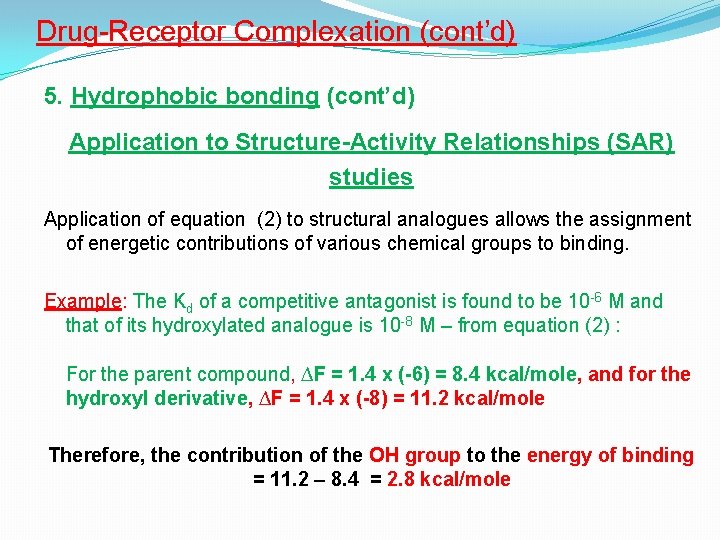
Drug-Receptor Complexation (cont’d) 5. Hydrophobic bonding (cont’d) Application to Structure-Activity Relationships (SAR) studies Application of equation (2) to structural analogues allows the assignment of energetic contributions of various chemical groups to binding. Example: The Kd of a competitive antagonist is found to be 10 -6 M and that of its hydroxylated analogue is 10 -8 M – from equation (2) : For the parent compound, ∆F = 1. 4 x (-6) = 8. 4 kcal/mole, and for the hydroxyl derivative, ∆F = 1. 4 x (-8) = 11. 2 kcal/mole Therefore, the contribution of the OH group to the energy of binding = 11. 2 – 8. 4 = 2. 8 kcal/mole
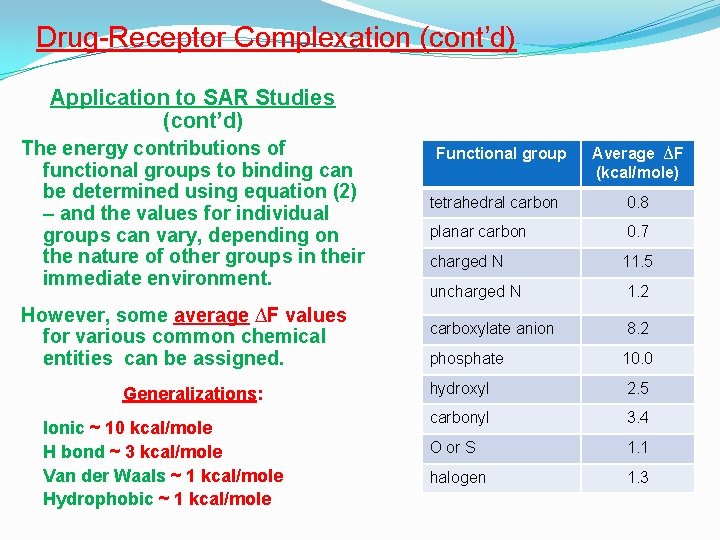
Drug-Receptor Complexation (cont’d) Application to SAR Studies (cont’d) The energy contributions of functional groups to binding can be determined using equation (2) – and the values for individual groups can vary, depending on the nature of other groups in their immediate environment. However, some average ∆F values for various common chemical entities can be assigned. Generalizations: Ionic ~ 10 kcal/mole H bond ~ 3 kcal/mole Van der Waals ~ 1 kcal/mole Hydrophobic ~ 1 kcal/mole Functional group Average ∆F (kcal/mole) tetrahedral carbon 0. 8 planar carbon 0. 7 charged N 11. 5 uncharged N 1. 2 carboxylate anion 8. 2 phosphate 10. 0 hydroxyl 2. 5 carbonyl 3. 4 O or S 1. 1 halogen 1. 3
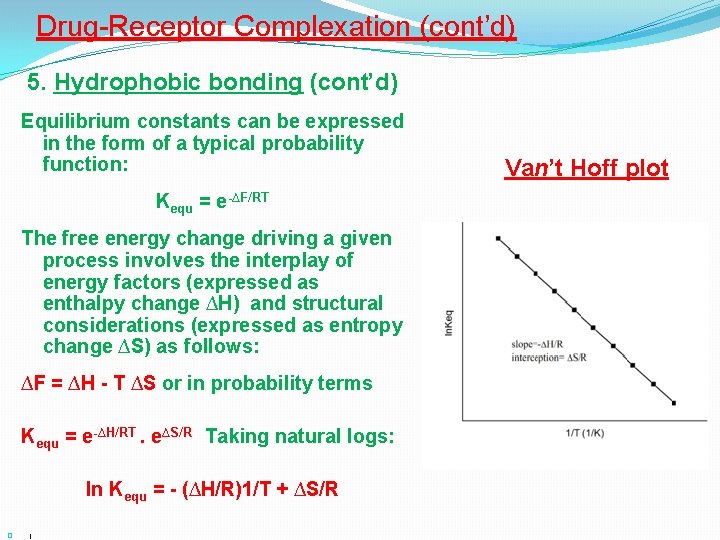
Drug-Receptor Complexation (cont’d) 5. Hydrophobic bonding (cont’d) Equilibrium constants can be expressed in the form of a typical probability function: Kequ = e-∆F/RT The free energy change driving a given process involves the interplay of energy factors (expressed as enthalpy change ∆H) and structural considerations (expressed as entropy change ∆S) as follows: ∆F = ∆H - T ∆S or in probability terms Kequ = e-∆H/RT. e∆S/R Taking natural logs: ln Kequ = - (∆H/R)1/T + ∆S/R � ( Van’t Hoff plot
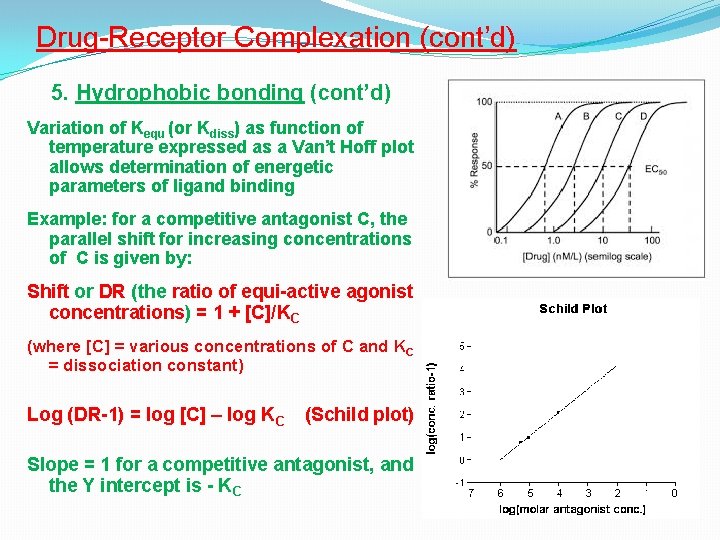
Drug-Receptor Complexation (cont’d) 5. Hydrophobic bonding (cont’d) Variation of Kequ (or Kdiss) as function of temperature expressed as a Van’t Hoff plot allows determination of energetic parameters of ligand binding Example: for a competitive antagonist C, the parallel shift for increasing concentrations of C is given by: Shift or DR (the ratio of equi-active agonist concentrations) = 1 + [C]/KC (where [C] = various concentrations of C and KC = dissociation constant) Log (DR-1) = log [C] – log KC (Schild plot) Slope = 1 for a competitive antagonist, and the Y intercept is - KC
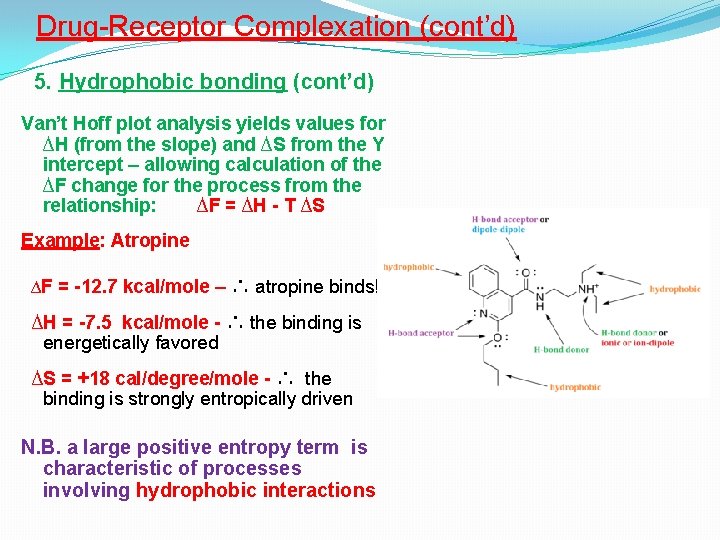
Drug-Receptor Complexation (cont’d) 5. Hydrophobic bonding (cont’d) Van’t Hoff plot analysis yields values for ∆H (from the slope) and ∆S from the Y intercept – allowing calculation of the ∆F change for the process from the relationship: ∆F = ∆H - T ∆S Example: Atropine ∆F = -12. 7 kcal/mole – ∴ atropine binds! ∆H = -7. 5 kcal/mole - ∴ the binding is energetically favored ∆S = +18 cal/degree/mole - ∴ the binding is strongly entropically driven N. B. a large positive entropy term is characteristic of processes involving hydrophobic interactions
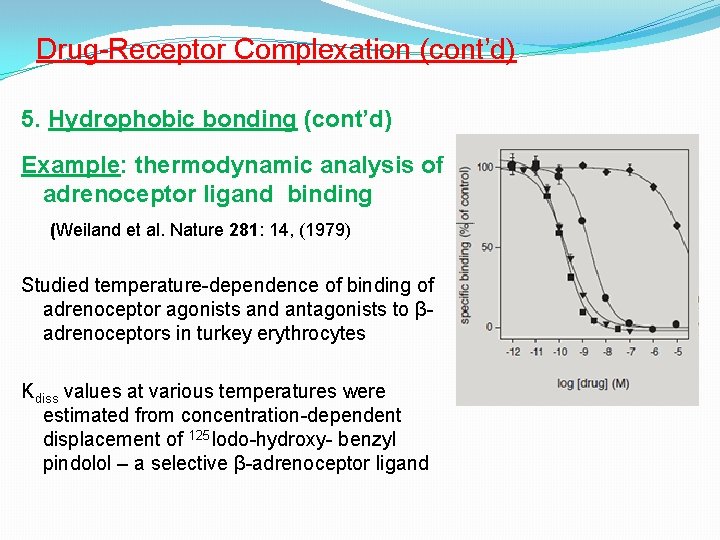
Drug-Receptor Complexation (cont’d) 5. Hydrophobic bonding (cont’d) Example: thermodynamic analysis of adrenoceptor ligand binding (Weiland et al. Nature 281: 14, (1979) Studied temperature-dependence of binding of adrenoceptor agonists and antagonists to βadrenoceptors in turkey erythrocytes Kdiss values at various temperatures were estimated from concentration-dependent displacement of 125 Iodo-hydroxy- benzyl pindolol – a selective β-adrenoceptor ligand
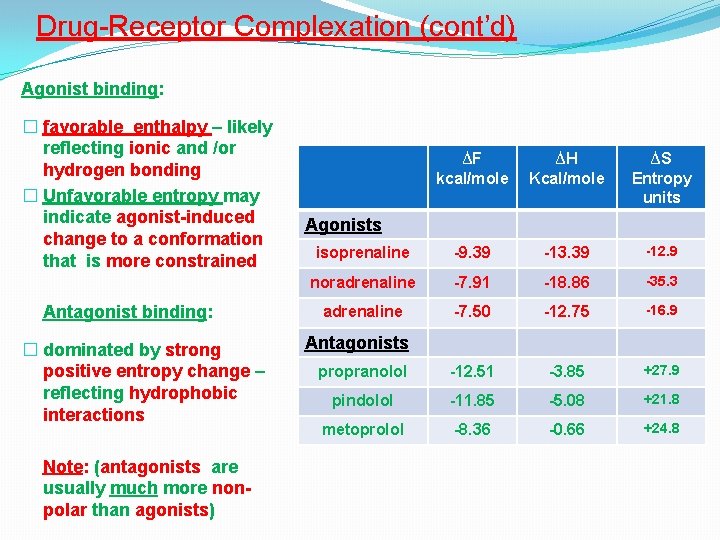
Drug-Receptor Complexation (cont’d) Agonist binding: � favorable enthalpy – likely reflecting ionic and /or hydrogen bonding � Unfavorable entropy may indicate agonist-induced change to a conformation that is more constrained Antagonist binding: � dominated by strong positive entropy change – reflecting hydrophobic interactions Note: (antagonists are usually much more nonpolar than agonists) ∆F kcal/mole ∆H Kcal/mole ∆S Entropy units isoprenaline -9. 39 -13. 39 -12. 9 noradrenaline -7. 91 -18. 86 -35. 3 adrenaline -7. 50 -12. 75 -16. 9 propranolol -12. 51 -3. 85 +27. 9 pindolol -11. 85 -5. 08 +21. 8 metoprolol -8. 36 -0. 66 +24. 8 Agonists Antagonists
200 + 200 = 400
Complexation reaction
Physicochemical properties of drugs
Dissolution coefficient
Aspects of responsibility
It encompasses several different aspects including
Psychological aspects of interlanguage
Dmtf cim
The 7 elements of culture
Ethical and legal issues in community health nursing
Quantitative chemistry grade 11
Ethical and professional issues in information security
It involves five aspects
Aspects of american dream
Aspects of organizational culture
Legal aspects of catering premises
Modelo cognitivo
Aspects of development in a country
Additional aspects of aqueous equilibria
Rod ellis 2003
Palmer hayden jeunesse
Crucial aspects of preparing digital audio files
Health aspects of family planning
Writing that is true and factual
Elements of setting
Legal aspects of software engineering
Medicolegal aspects of pregnancy
Psycholinguistic aspects of interlanguage
Aspects of language lesson 1
Involves managing all aspects of a customer relationship
Aspects of systems thinking
Legal aspects of advertising
Stylistic continuum in interlanguage
Aspects of attitude
Behavioral aspects of management control
6 aspects of wellness
Legal and ethical aspects of nursing chapter 3