OPTIC 2013 Simulation of LightEmitting Diodes and Solar
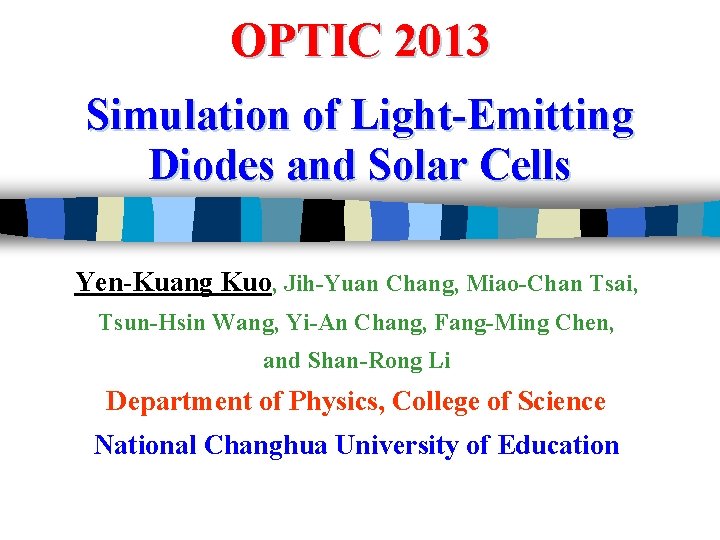
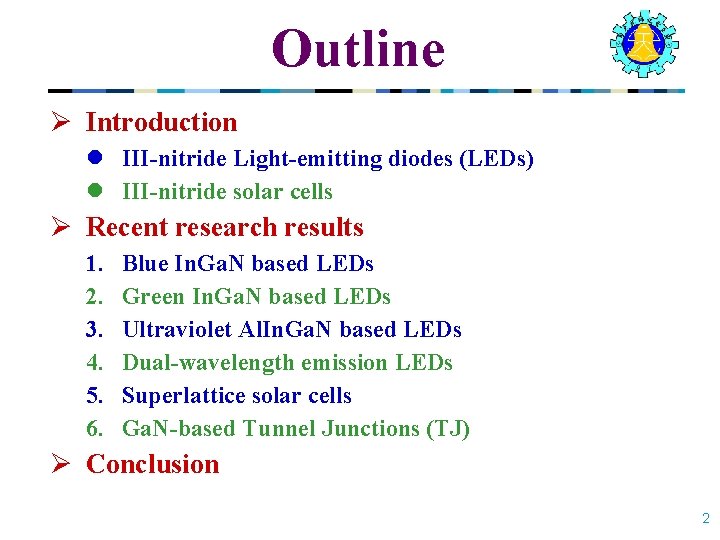
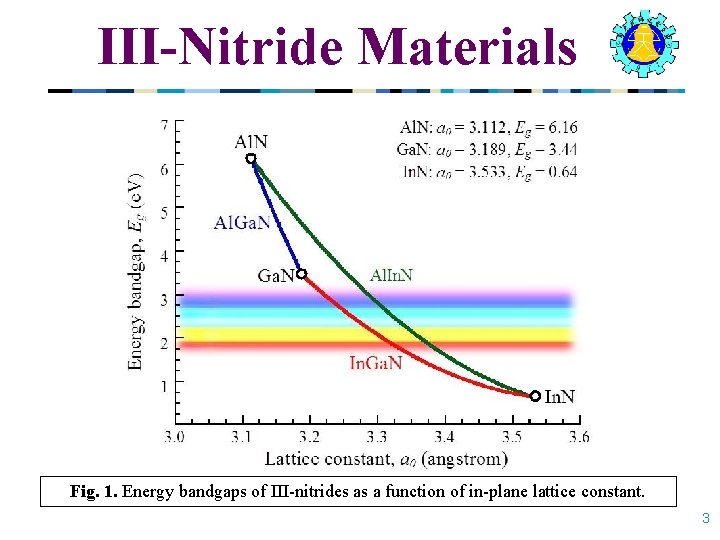
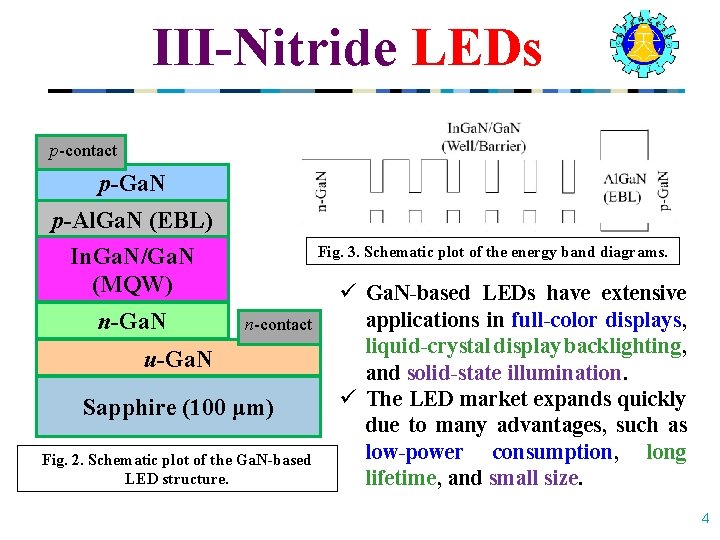
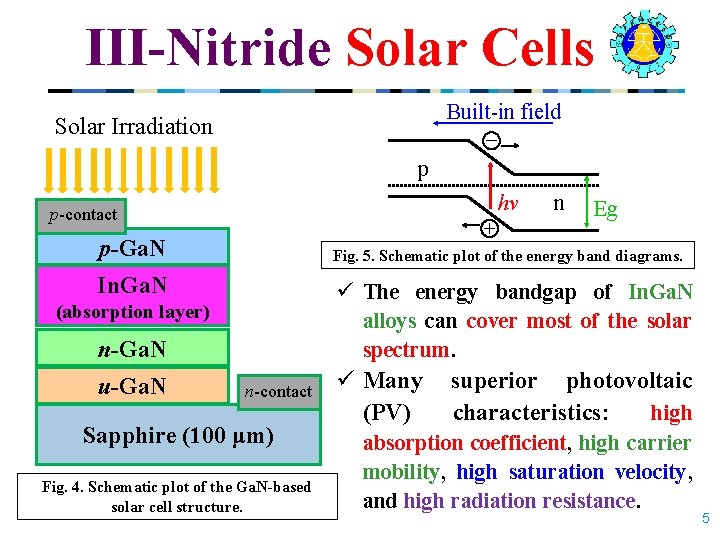
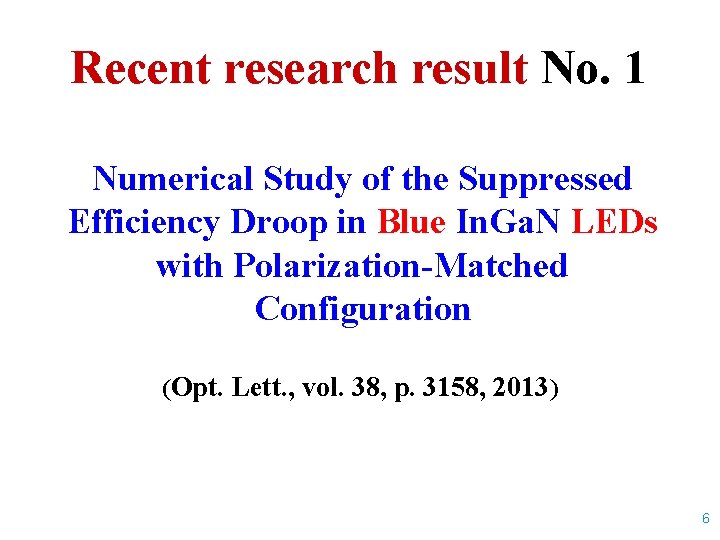
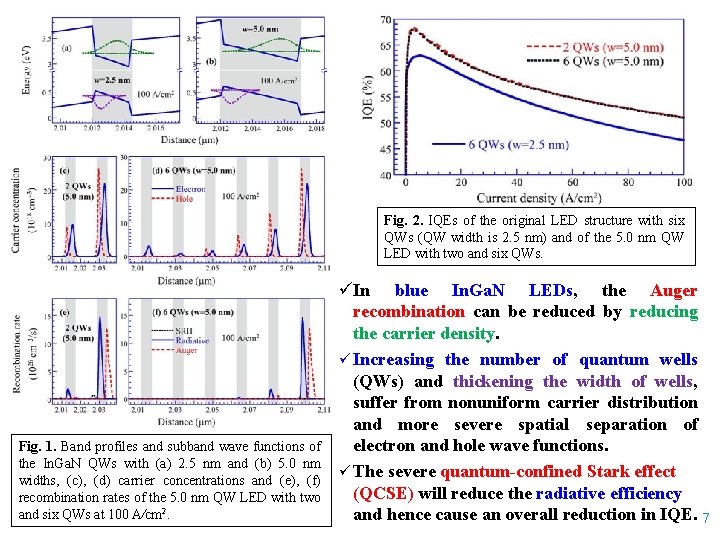
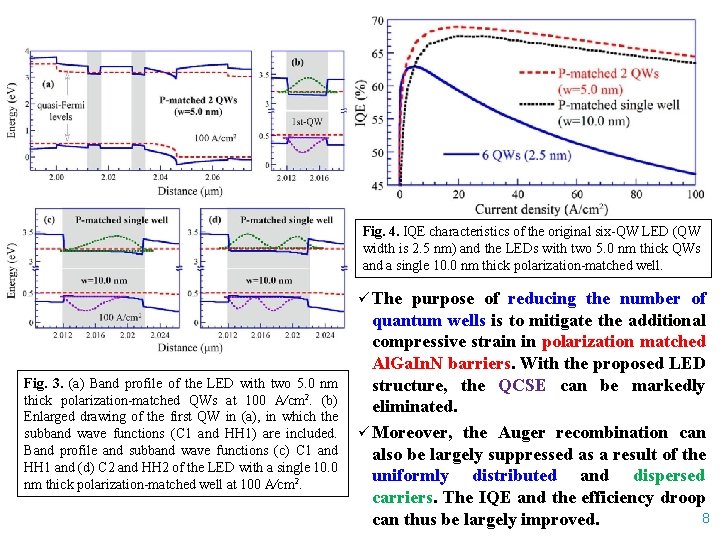
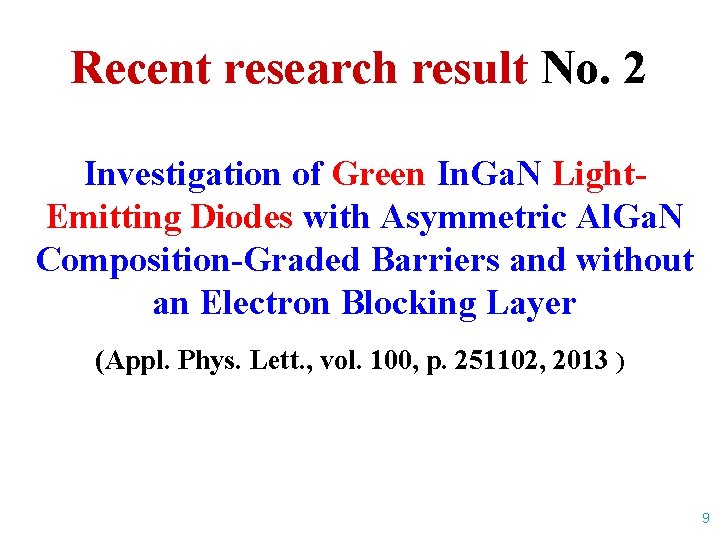
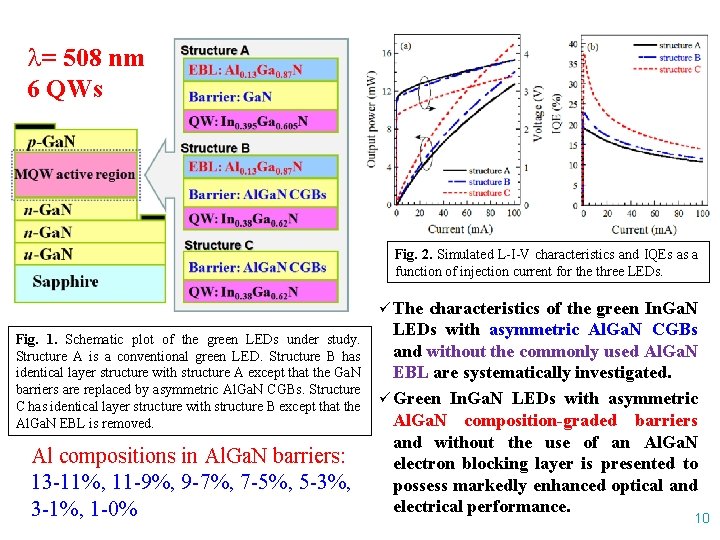
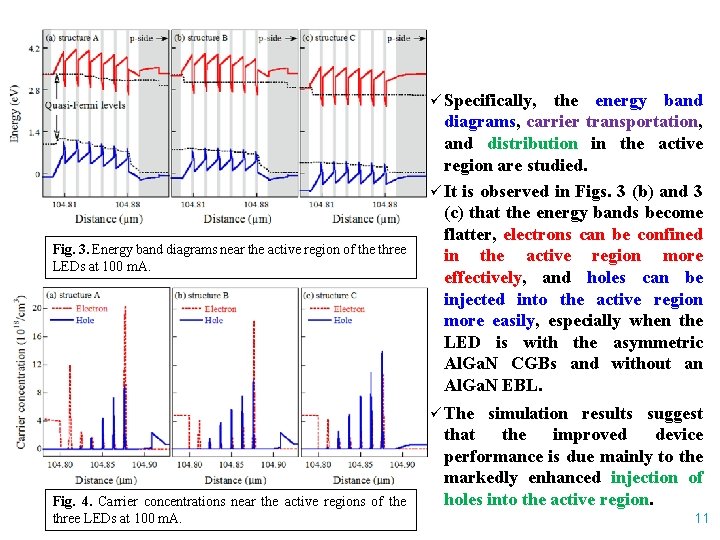
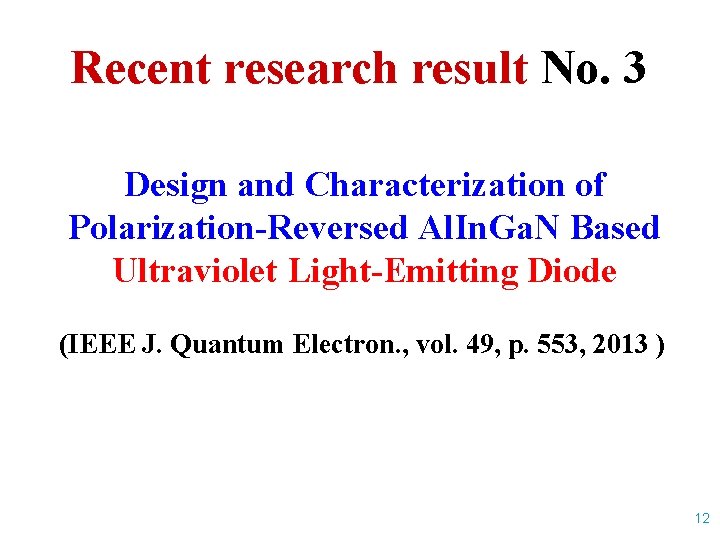
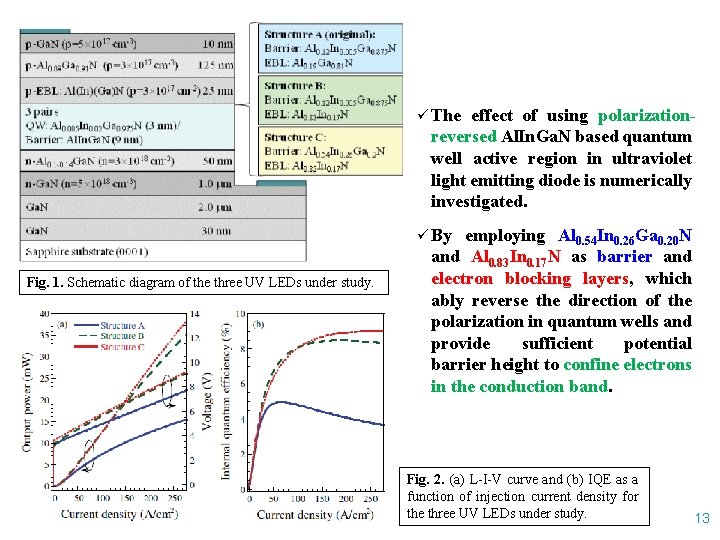
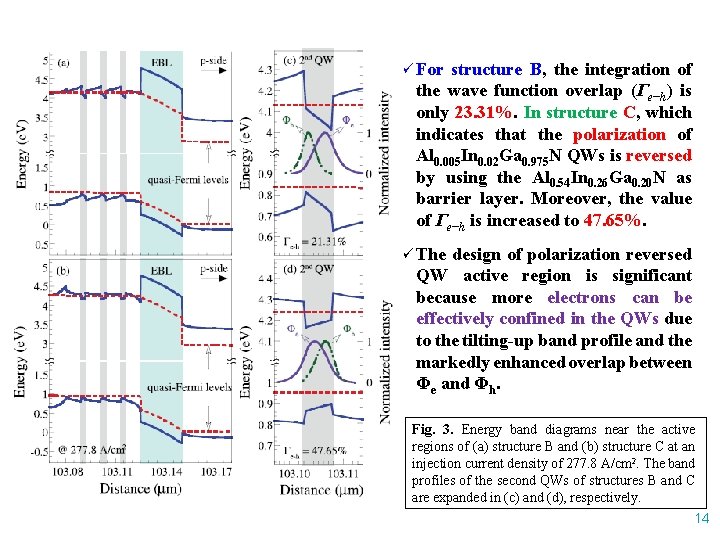
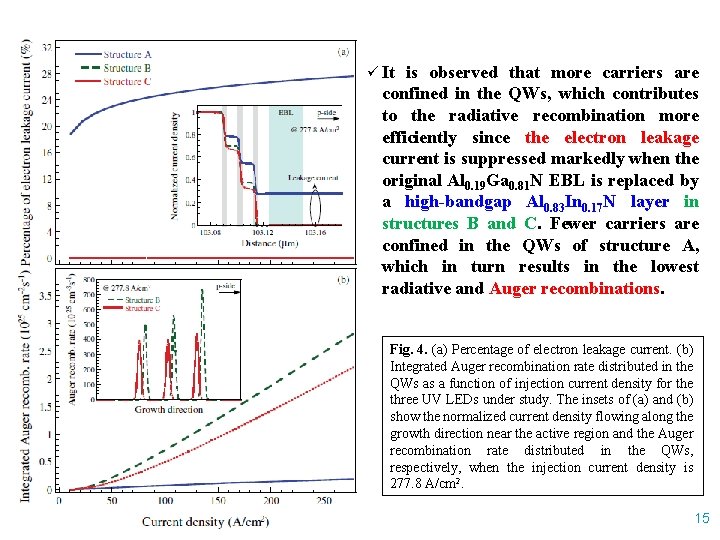
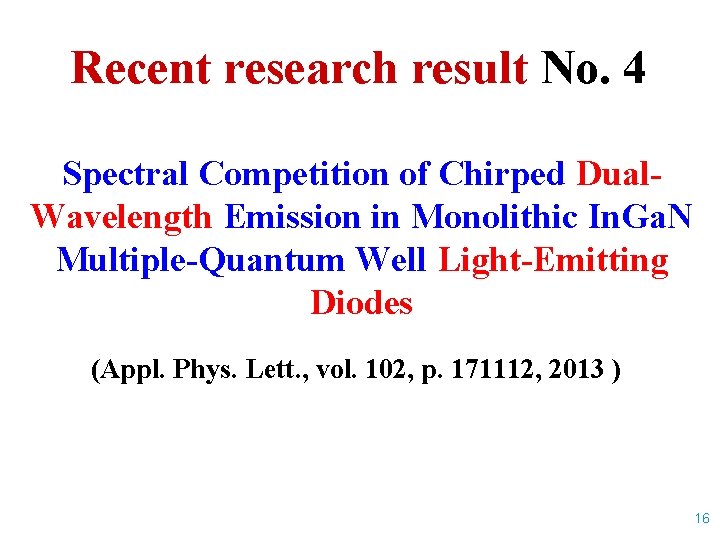
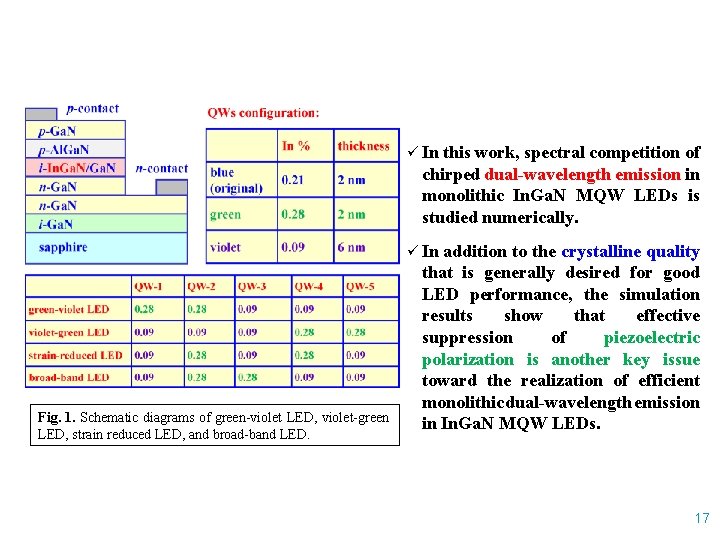
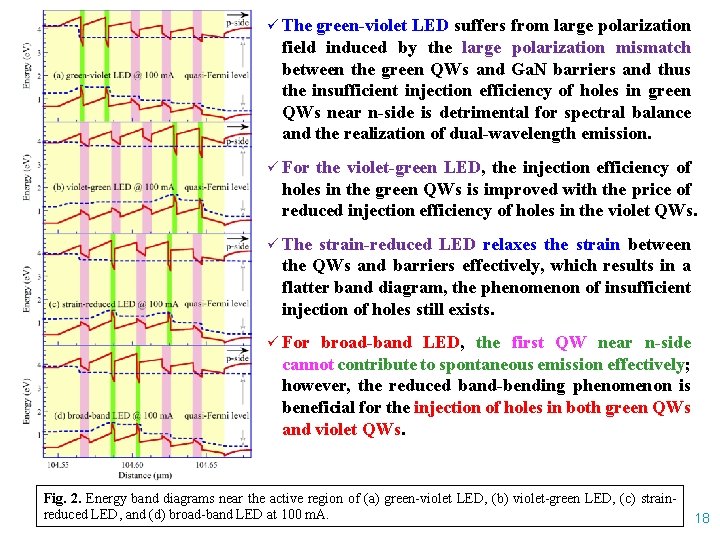
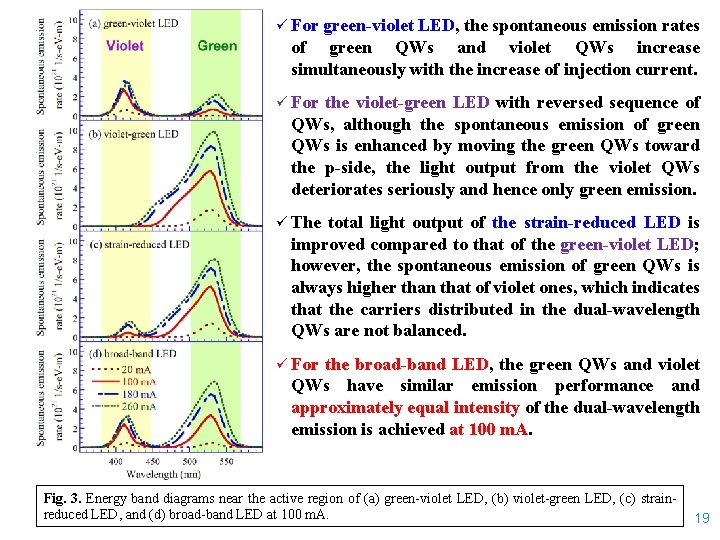
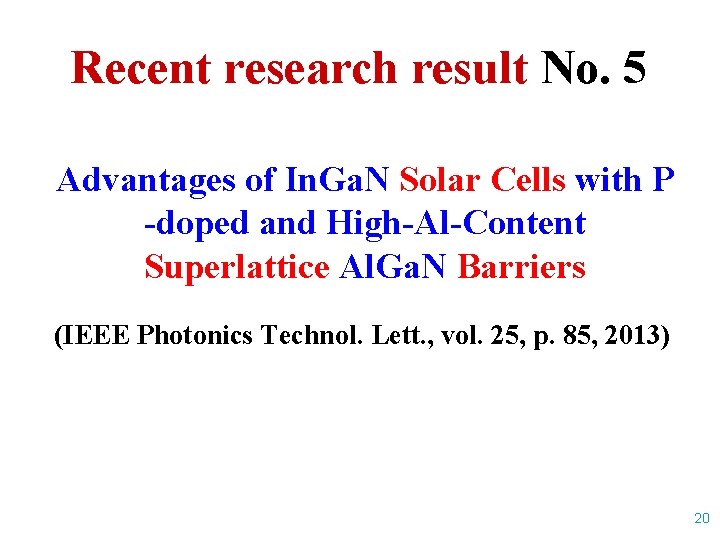
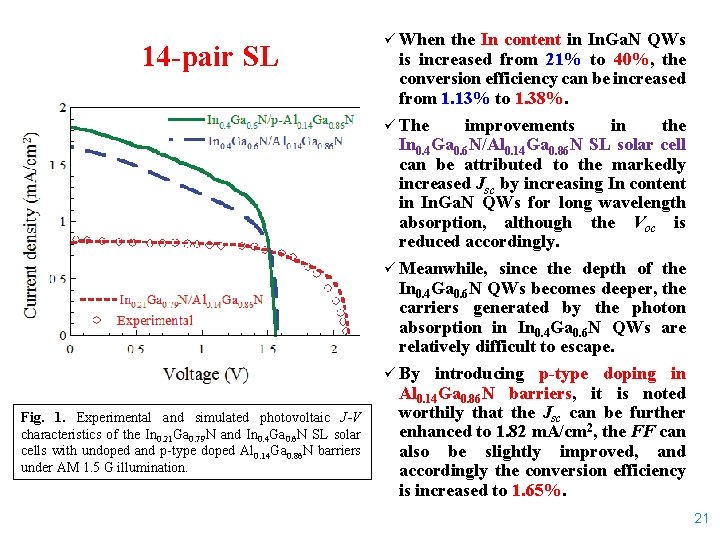
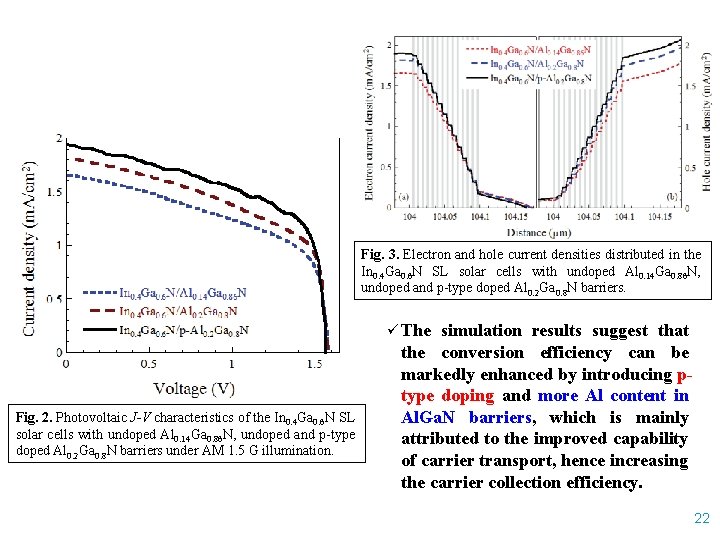
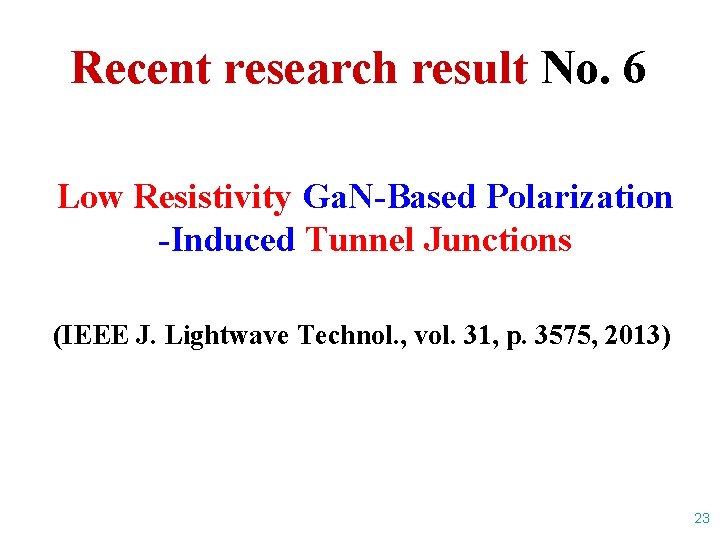
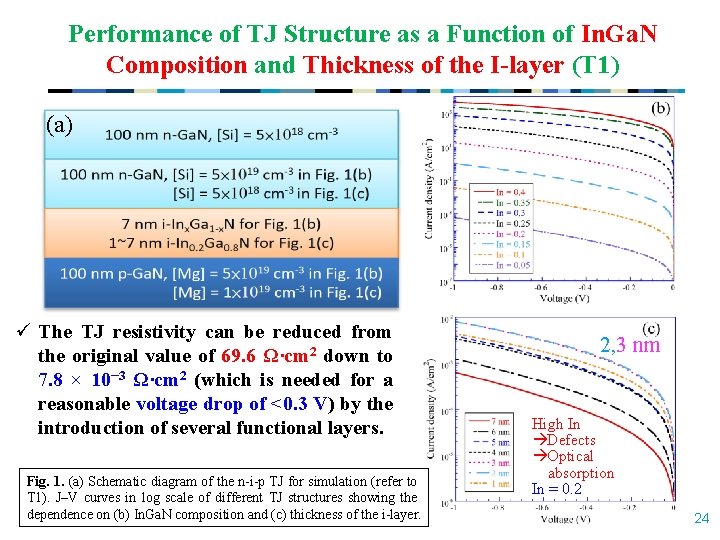
![Dependence on [Mg] and [Si] Doping Densities (T 2) (a) ü Firstly, inserting two Dependence on [Mg] and [Si] Doping Densities (T 2) (a) ü Firstly, inserting two](https://slidetodoc.com/presentation_image_h2/d322b50bc80c8e7ce92697e89a1656ee/image-25.jpg)
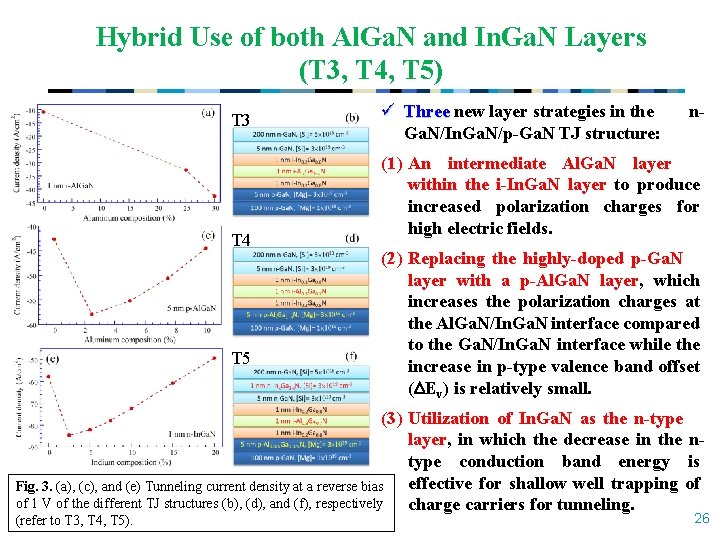
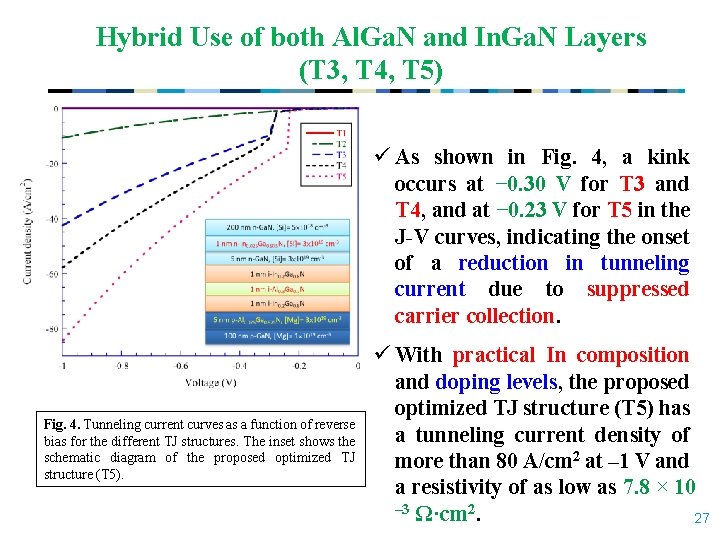
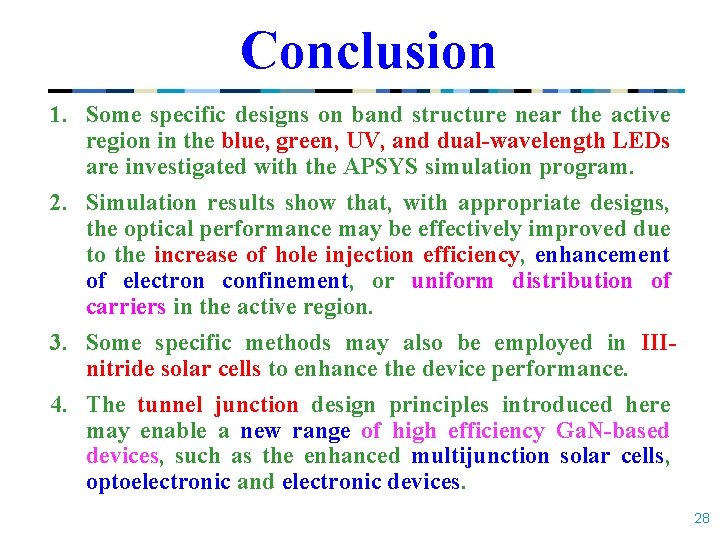
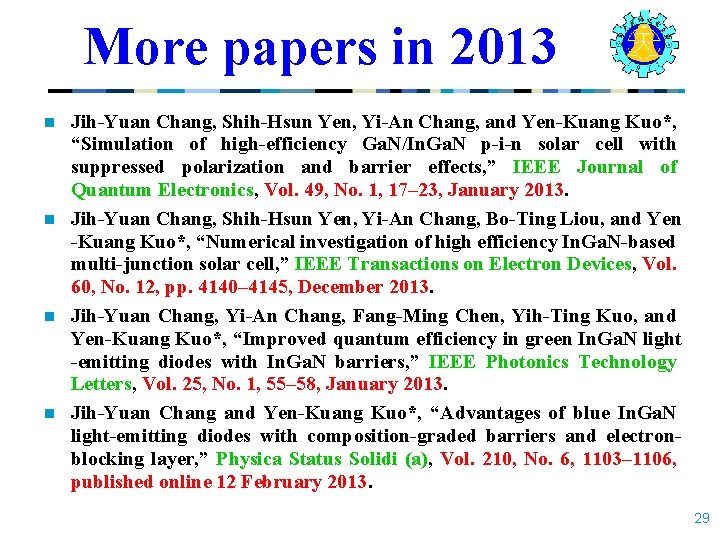
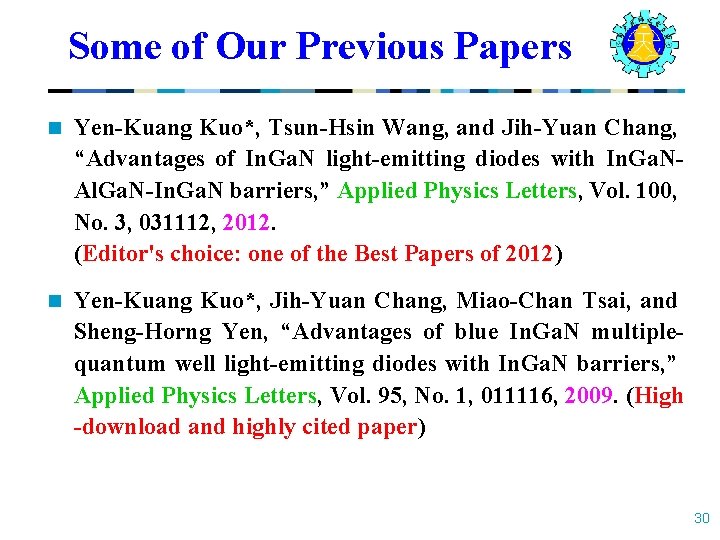
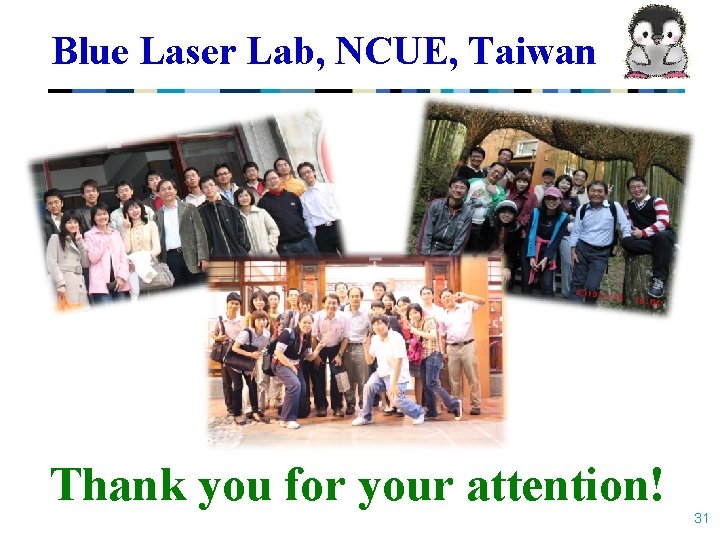
- Slides: 31
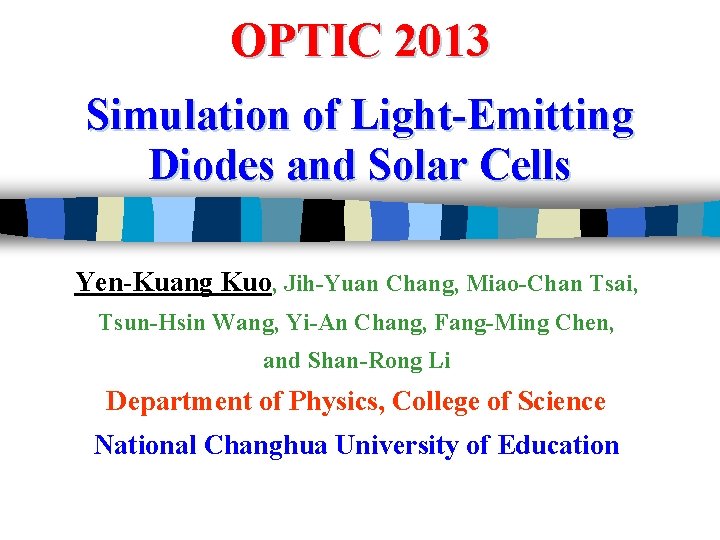
OPTIC 2013 Simulation of Light-Emitting Diodes and Solar Cells Yen-Kuang Kuo, Jih-Yuan Chang, Miao-Chan Tsai, Tsun-Hsin Wang, Yi-An Chang, Fang-Ming Chen, and Shan-Rong Li Department of Physics, College of Science National Changhua University of Education 1
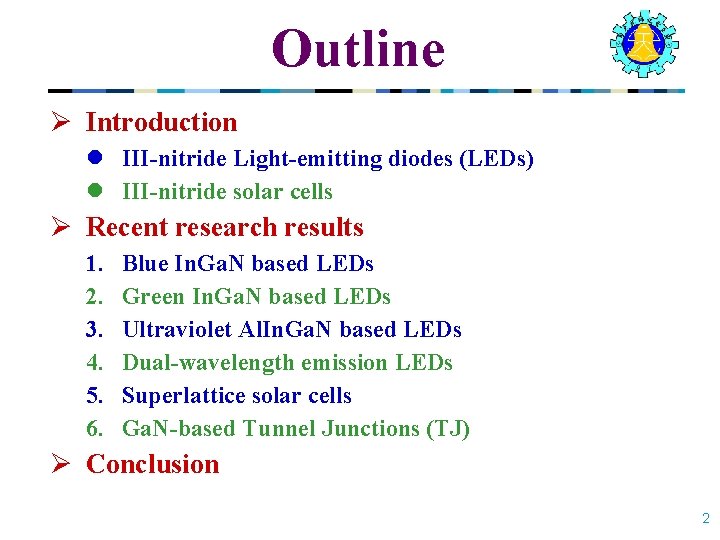
Outline Ø Introduction l III-nitride Light-emitting diodes (LEDs) l III-nitride solar cells Ø Recent research results 1. 2. 3. 4. 5. 6. Blue In. Ga. N based LEDs Green In. Ga. N based LEDs Ultraviolet Al. In. Ga. N based LEDs Dual-wavelength emission LEDs Superlattice solar cells Ga. N-based Tunnel Junctions (TJ) Ø Conclusion 2
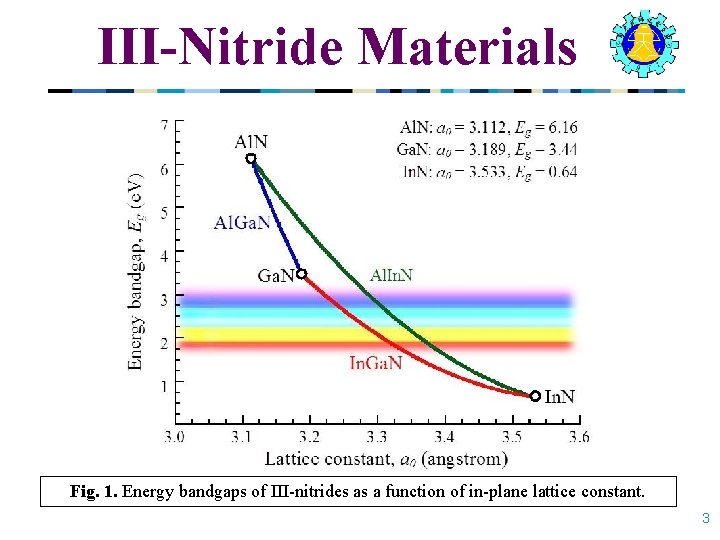
III-Nitride Materials Fig. 1. Energy bandgaps of III-nitrides as a function of in-plane lattice constant. 3
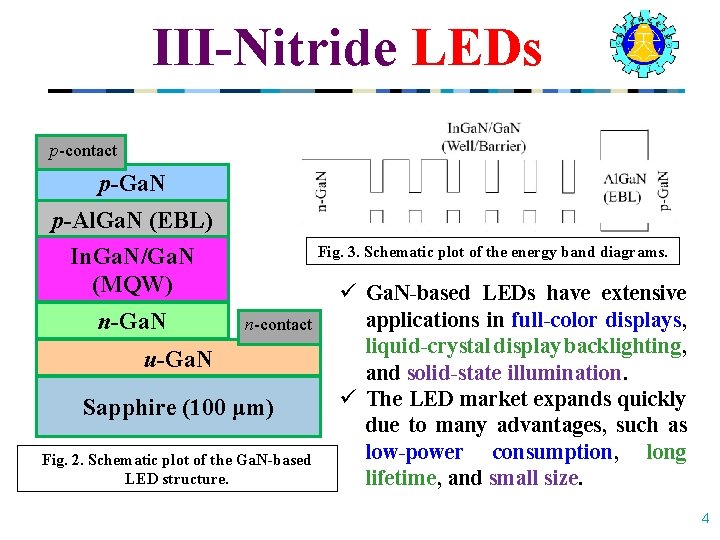
III-Nitride LEDs p-contact p-Ga. N p-Al. Ga. N (EBL) In. Ga. N/Ga. N (MQW) n-Ga. N Fig. 3. Schematic plot of the energy band diagrams. n-contact u-Ga. N Sapphire (100 μm) Fig. 2. Schematic plot of the Ga. N-based LED structure. ü Ga. N-based LEDs have extensive applications in full-color displays, liquid-crystal display backlighting, and solid-state illumination. ü The LED market expands quickly due to many advantages, such as low-power consumption, long lifetime, and small size. 4
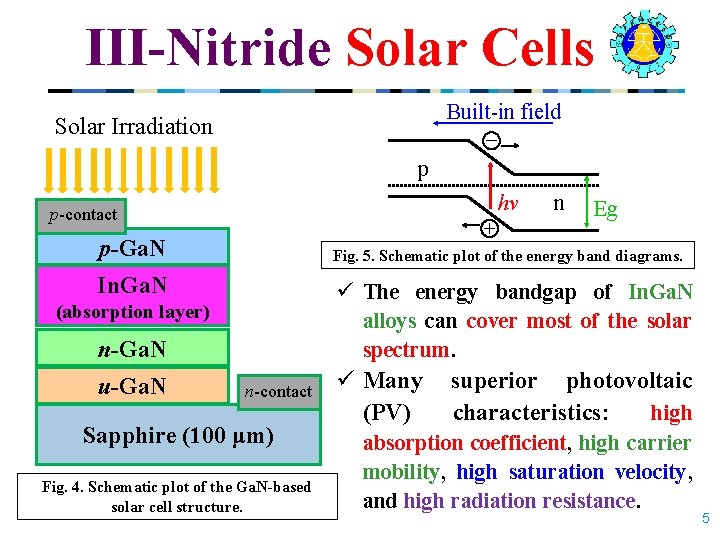
III-Nitride Solar Cells Built-in field – Solar Irradiation p hν p-contact + p-Ga. N Eg Fig. 5. Schematic plot of the energy band diagrams. In. Ga. N ü The energy bandgap of In. Ga. N alloys can cover most of the solar spectrum. (absorption layer) n-Ga. N u-Ga. N n n-contact Sapphire (100 μm) Fig. 4. Schematic plot of the Ga. N-based solar cell structure. ü Many (PV) superior photovoltaic characteristics: high absorption coefficient, high carrier mobility, high saturation velocity, and high radiation resistance. 5
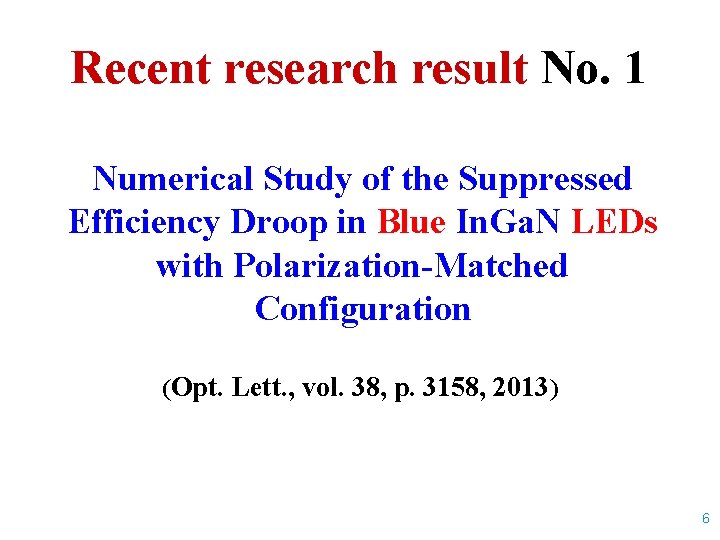
Recent research result No. 1 Numerical Study of the Suppressed Efficiency Droop in Blue In. Ga. N LEDs with Polarization-Matched Configuration (Opt. Lett. , vol. 38, p. 3158, 2013) 6
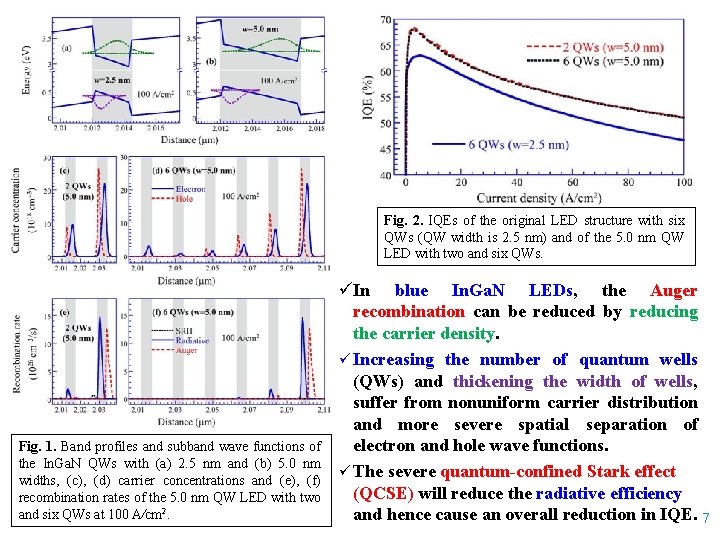
Fig. 2. IQEs of the original LED structure with six QWs (QW width is 2. 5 nm) and of the 5. 0 nm QW LED with two and six QWs. Fig. 1. Band profiles and subband wave functions of the In. Ga. N QWs with (a) 2. 5 nm and (b) 5. 0 nm widths, (c), (d) carrier concentrations and (e), (f) recombination rates of the 5. 0 nm QW LED with two and six QWs at 100 A∕cm 2. üIn blue In. Ga. N LEDs, the Auger recombination can be reduced by reducing the carrier density. ü Increasing the number of quantum wells (QWs) and thickening the width of wells, suffer from nonuniform carrier distribution and more severe spatial separation of electron and hole wave functions. ü The severe quantum-confined Stark effect (QCSE) will reduce the radiative efficiency and hence cause an overall reduction in IQE. 7
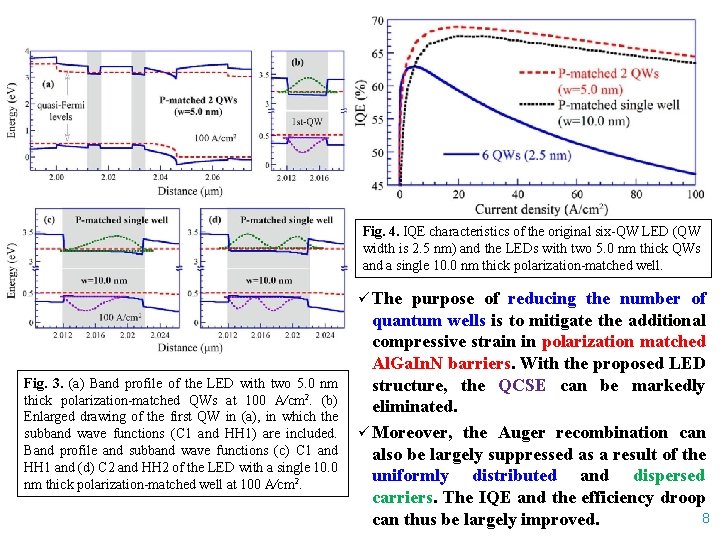
Fig. 4. IQE characteristics of the original six-QW LED (QW width is 2. 5 nm) and the LEDs with two 5. 0 nm thick QWs and a single 10. 0 nm thick polarization-matched well. ü The Fig. 3. (a) Band profile of the LED with two 5. 0 nm thick polarization-matched QWs at 100 A∕cm 2. (b) Enlarged drawing of the first QW in (a), in which the subband wave functions (C 1 and HH 1) are included. Band profile and subband wave functions (c) C 1 and HH 1 and (d) C 2 and HH 2 of the LED with a single 10. 0 nm thick polarization-matched well at 100 A∕cm 2. purpose of reducing the number of quantum wells is to mitigate the additional compressive strain in polarization matched Al. Ga. In. N barriers. With the proposed LED structure, the QCSE can be markedly eliminated. ü Moreover, the Auger recombination can also be largely suppressed as a result of the uniformly distributed and dispersed carriers. The IQE and the efficiency droop 8 can thus be largely improved.
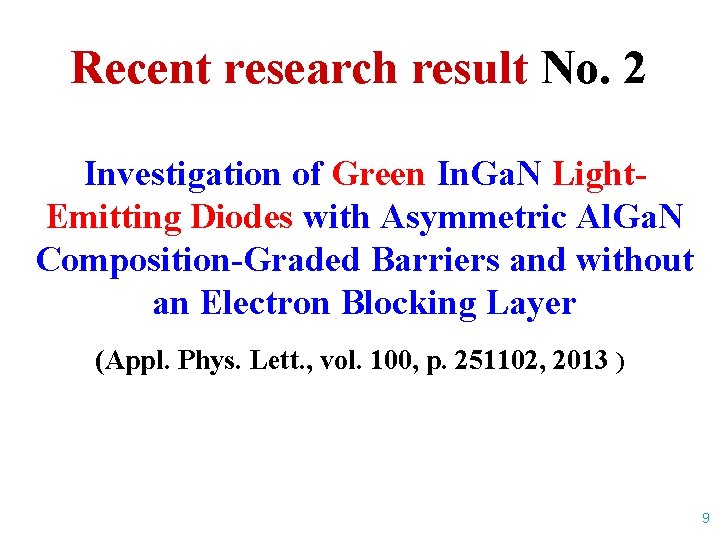
Recent research result No. 2 Investigation of Green In. Ga. N Light. Emitting Diodes with Asymmetric Al. Ga. N Composition-Graded Barriers and without an Electron Blocking Layer (Appl. Phys. Lett. , vol. 100, p. 251102, 2013 ) 9
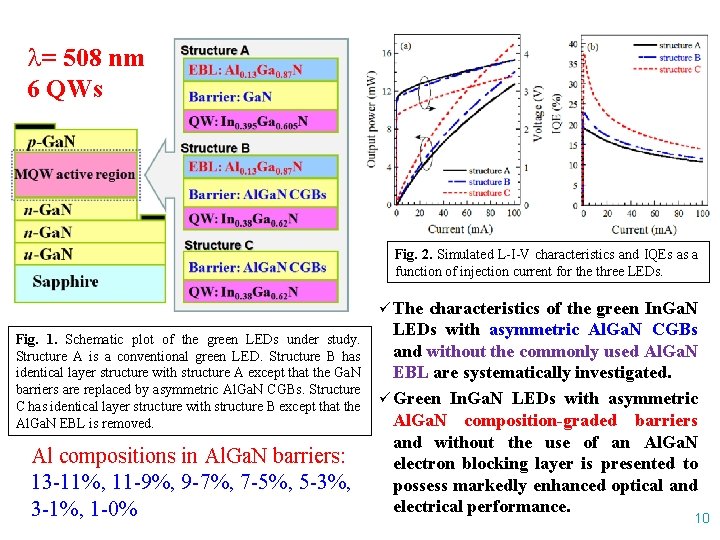
l= 508 nm 6 QWs Fig. 2. Simulated L-I-V characteristics and IQEs as a function of injection current for the three LEDs. ü The Fig. 1. Schematic plot of the green LEDs under study. Structure A is a conventional green LED. Structure B has identical layer structure with structure A except that the Ga. N barriers are replaced by asymmetric Al. Ga. N CGBs. Structure C has identical layer structure with structure B except that the Al. Ga. N EBL is removed. Al compositions in Al. Ga. N barriers: 13 -11%, 11 -9%, 9 -7%, 7 -5%, 5 -3%, 3 -1%, 1 -0% characteristics of the green In. Ga. N LEDs with asymmetric Al. Ga. N CGBs and without the commonly used Al. Ga. N EBL are systematically investigated. ü Green In. Ga. N LEDs with asymmetric Al. Ga. N composition-graded barriers and without the use of an Al. Ga. N electron blocking layer is presented to possess markedly enhanced optical and electrical performance. 10
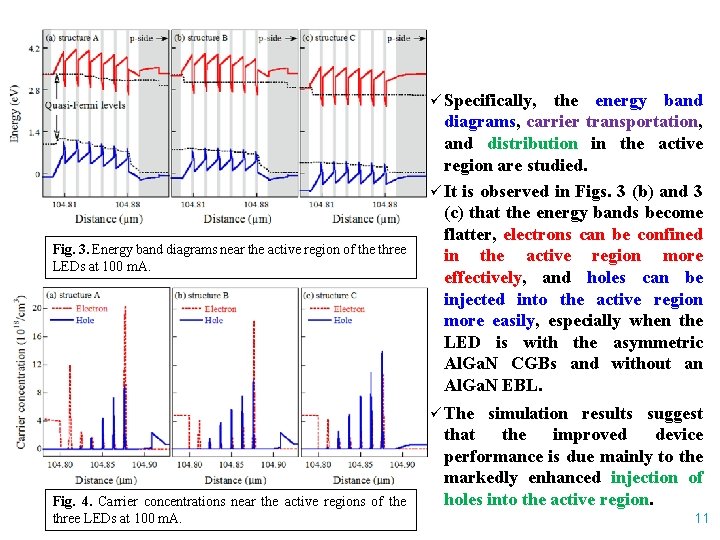
ü Specifically, Fig. 3. Energy band diagrams near the active region of the three LEDs at 100 m. A. the energy band diagrams, carrier transportation, and distribution in the active region are studied. ü It is observed in Figs. 3 (b) and 3 (c) that the energy bands become flatter, electrons can be confined in the active region more effectively, and holes can be injected into the active region more easily, especially when the LED is with the asymmetric Al. Ga. N CGBs and without an Al. Ga. N EBL. ü The Fig. 4. Carrier concentrations near the active regions of the three LEDs at 100 m. A. simulation results suggest that the improved device performance is due mainly to the markedly enhanced injection of holes into the active region. 11
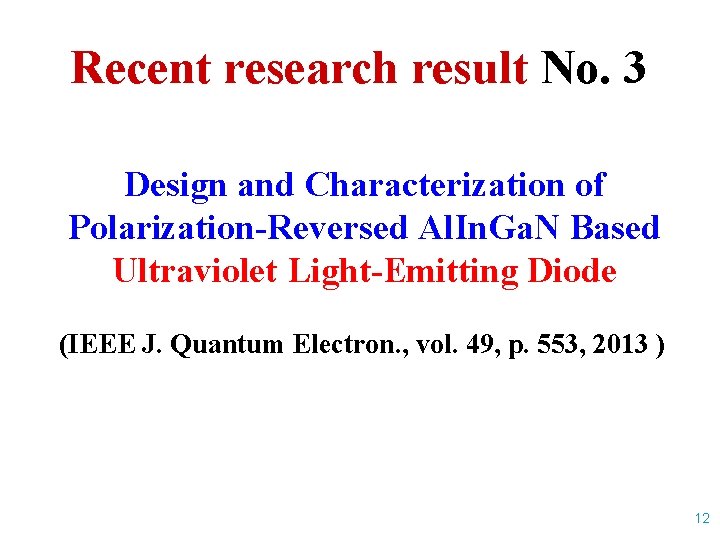
Recent research result No. 3 Design and Characterization of Polarization-Reversed Al. In. Ga. N Based Ultraviolet Light-Emitting Diode (IEEE J. Quantum Electron. , vol. 49, p. 553, 2013 ) 12
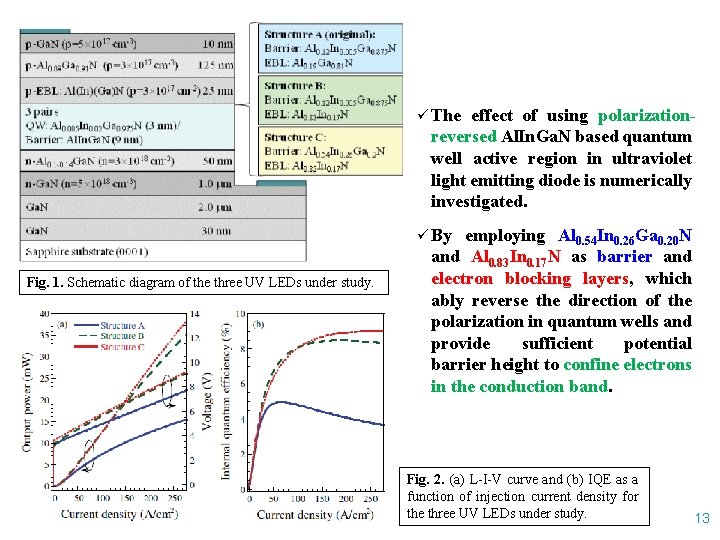
ü The effect of using polarizationreversed Al. In. Ga. N based quantum well active region in ultraviolet light emitting diode is numerically investigated. ü By Fig. 1. Schematic diagram of the three UV LEDs under study. employing Al 0. 54 In 0. 26 Ga 0. 20 N and Al 0. 83 In 0. 17 N as barrier and electron blocking layers, which ably reverse the direction of the polarization in quantum wells and provide sufficient potential barrier height to confine electrons in the conduction band. Fig. 2. (a) L-I-V curve and (b) IQE as a function of injection current density for the three UV LEDs under study. 13
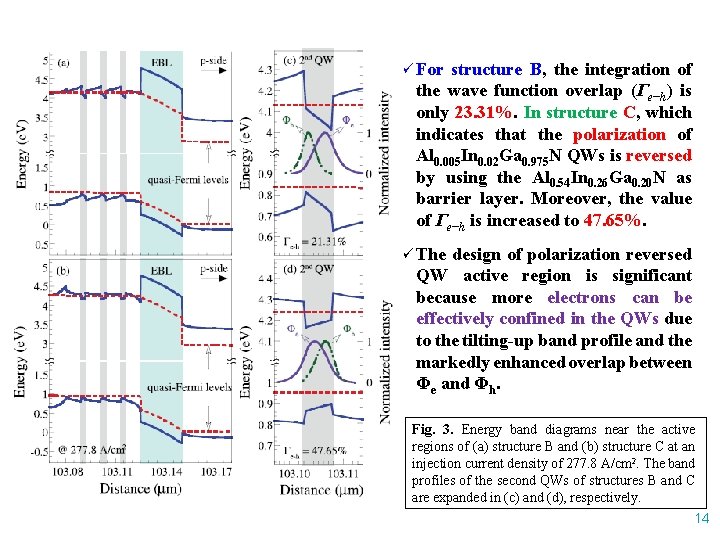
ü For structure B, the integration of the wave function overlap (Γe−h) is only 23. 31%. In structure C, which indicates that the polarization of Al 0. 005 In 0. 02 Ga 0. 975 N QWs is reversed by using the Al 0. 54 In 0. 26 Ga 0. 20 N as barrier layer. Moreover, the value of Γe−h is increased to 47. 65%. ü The design of polarization reversed QW active region is significant because more electrons can be effectively confined in the QWs due to the tilting-up band profile and the markedly enhanced overlap between Φe and Φh. Fig. 3. Energy band diagrams near the active regions of (a) structure B and (b) structure C at an injection current density of 277. 8 A/cm 2. The band profiles of the second QWs of structures B and C are expanded in (c) and (d), respectively. 14
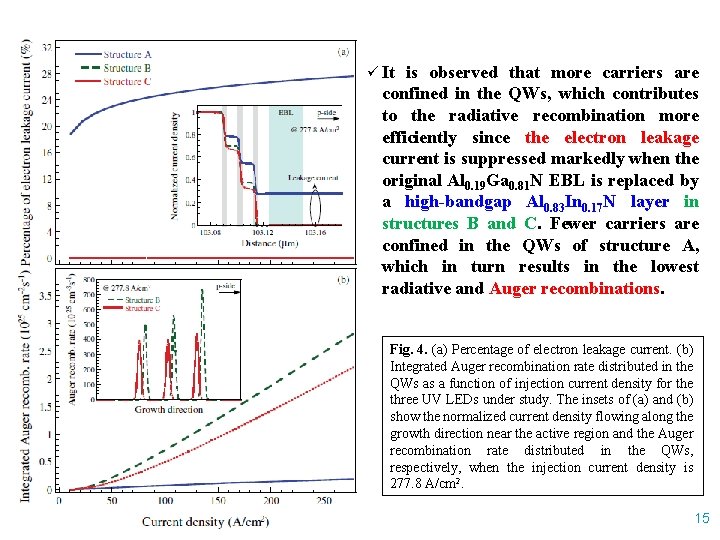
ü It is observed that more carriers are confined in the QWs, which contributes to the radiative recombination more efficiently since the electron leakage current is suppressed markedly when the original Al 0. 19 Ga 0. 81 N EBL is replaced by a high-bandgap Al 0. 83 In 0. 17 N layer in structures B and C. Fewer carriers are confined in the QWs of structure A, which in turn results in the lowest radiative and Auger recombinations. Fig. 4. (a) Percentage of electron leakage current. (b) Integrated Auger recombination rate distributed in the QWs as a function of injection current density for the three UV LEDs under study. The insets of (a) and (b) show the normalized current density flowing along the growth direction near the active region and the Auger recombination rate distributed in the QWs, respectively, when the injection current density is 277. 8 A/cm 2. 15
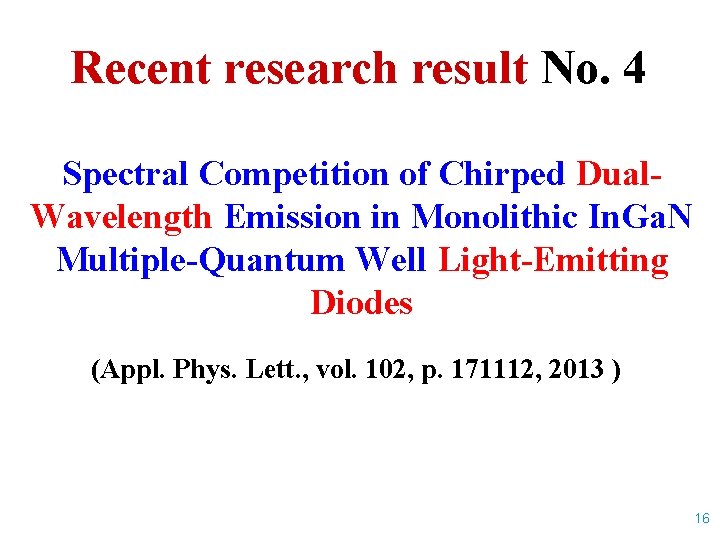
Recent research result No. 4 Spectral Competition of Chirped Dual. Wavelength Emission in Monolithic In. Ga. N Multiple-Quantum Well Light-Emitting Diodes (Appl. Phys. Lett. , vol. 102, p. 171112, 2013 ) 16
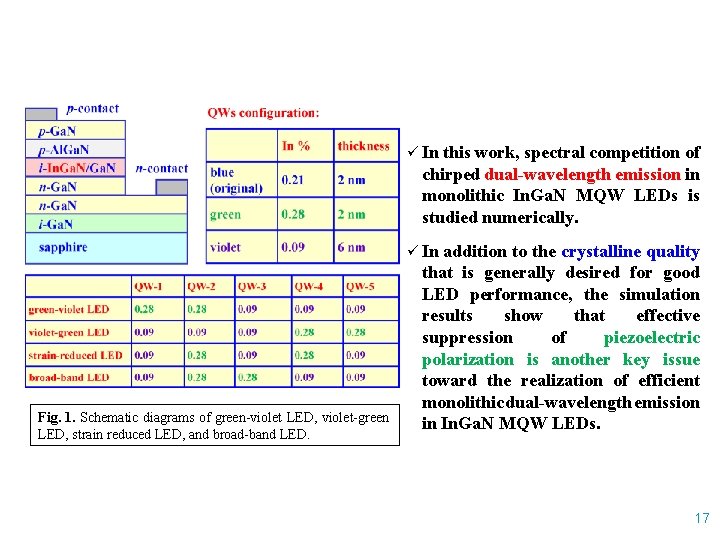
ü In this work, spectral competition of chirped dual-wavelength emission in monolithic In. Ga. N MQW LEDs is studied numerically. ü In Fig. 1. Schematic diagrams of green-violet LED, violet-green LED, strain reduced LED, and broad-band LED. addition to the crystalline quality that is generally desired for good LED performance, the simulation results show that effective suppression of piezoelectric polarization is another key issue toward the realization of efficient monolithic dual-wavelength emission in In. Ga. N MQW LEDs. 17
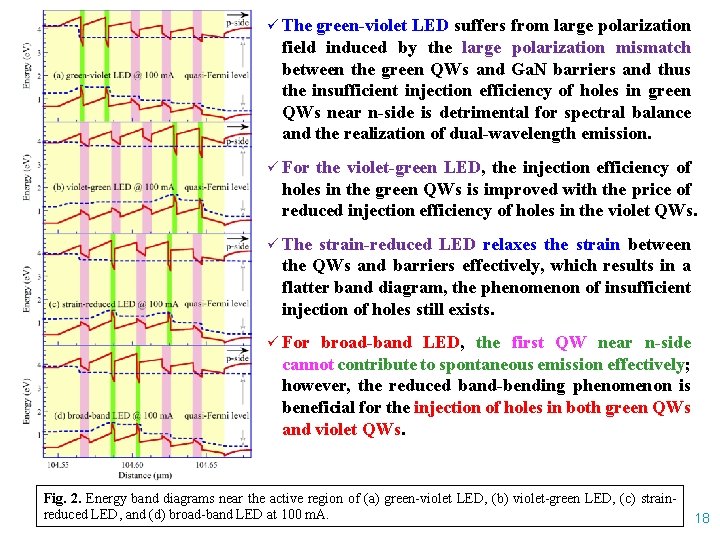
ü The green-violet LED suffers from large polarization field induced by the large polarization mismatch between the green QWs and Ga. N barriers and thus the insufficient injection efficiency of holes in green QWs near n-side is detrimental for spectral balance and the realization of dual-wavelength emission. ü For the violet-green LED, the injection efficiency of holes in the green QWs is improved with the price of reduced injection efficiency of holes in the violet QWs. ü The strain-reduced LED relaxes the strain between the QWs and barriers effectively, which results in a flatter band diagram, the phenomenon of insufficient injection of holes still exists. ü For broad-band LED, the first QW near n-side cannot contribute to spontaneous emission effectively; however, the reduced band-bending phenomenon is beneficial for the injection of holes in both green QWs and violet QWs. Fig. 2. Energy band diagrams near the active region of (a) green-violet LED, (b) violet-green LED, (c) strainreduced LED, and (d) broad-band LED at 100 m. A. 18
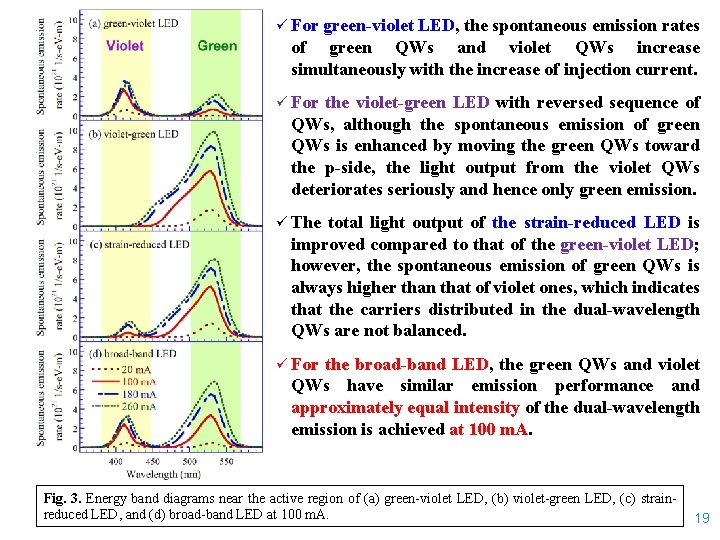
ü For green-violet LED, the spontaneous emission rates of green QWs and violet QWs increase simultaneously with the increase of injection current. ü For the violet-green LED with reversed sequence of QWs, although the spontaneous emission of green QWs is enhanced by moving the green QWs toward the p-side, the light output from the violet QWs deteriorates seriously and hence only green emission. ü The total light output of the strain-reduced LED is improved compared to that of the green-violet LED; however, the spontaneous emission of green QWs is always higher than that of violet ones, which indicates that the carriers distributed in the dual-wavelength QWs are not balanced. ü For the broad-band LED, the green QWs and violet QWs have similar emission performance and approximately equal intensity of the dual-wavelength emission is achieved at 100 m. A. Fig. 3. Energy band diagrams near the active region of (a) green-violet LED, (b) violet-green LED, (c) strainreduced LED, and (d) broad-band LED at 100 m. A. 19
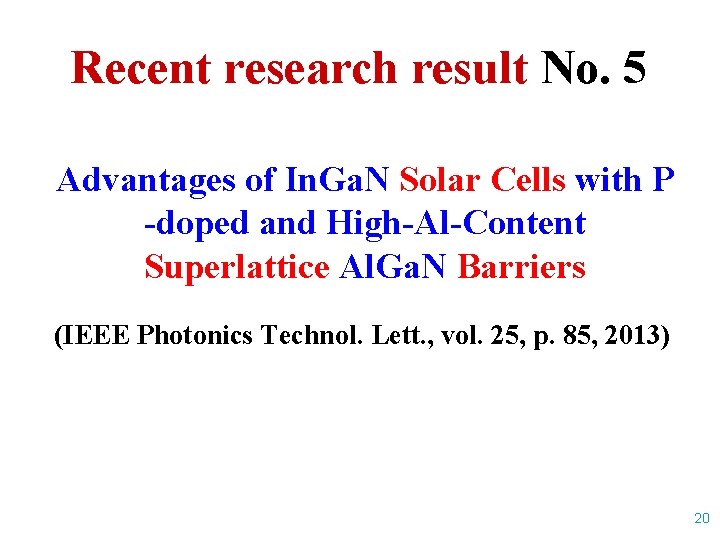
Recent research result No. 5 Advantages of In. Ga. N Solar Cells with P -doped and High-Al-Content Superlattice Al. Ga. N Barriers (IEEE Photonics Technol. Lett. , vol. 25, p. 85, 2013) 20
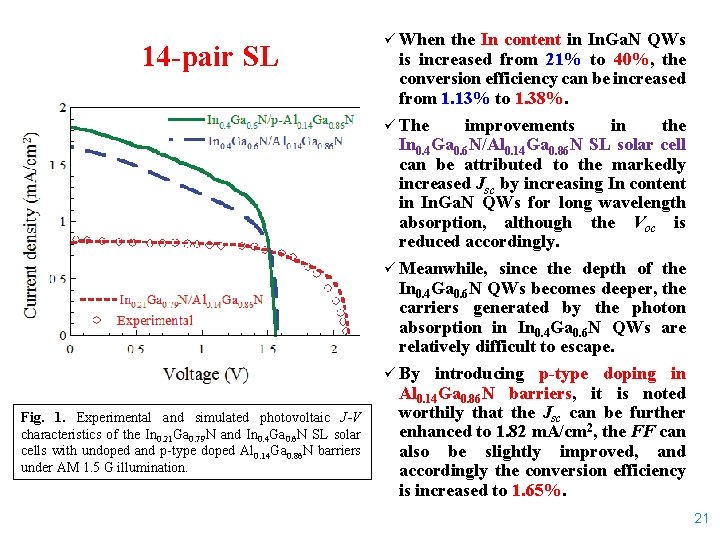
14 -pair SL Fig. 1. Experimental and simulated photovoltaic J-V characteristics of the In 0. 21 Ga 0. 79 N and In 0. 4 Ga 0. 6 N SL solar cells with undoped and p-type doped Al 0. 14 Ga 0. 86 N barriers under AM 1. 5 G illumination. ü When the In content in In. Ga. N QWs is increased from 21% to 40%, the conversion efficiency can be increased from 1. 13% to 1. 38%. ü The improvements in the In 0. 4 Ga 0. 6 N/Al 0. 14 Ga 0. 86 N SL solar cell can be attributed to the markedly increased Jsc by increasing In content in In. Ga. N QWs for long wavelength absorption, although the Voc is reduced accordingly. ü Meanwhile, since the depth of the In 0. 4 Ga 0. 6 N QWs becomes deeper, the carriers generated by the photon absorption in In 0. 4 Ga 0. 6 N QWs are relatively difficult to escape. ü By introducing p-type doping in Al 0. 14 Ga 0. 86 N barriers, it is noted worthily that the Jsc can be further enhanced to 1. 82 m. A/cm 2, the FF can also be slightly improved, and accordingly the conversion efficiency is increased to 1. 65%. 21
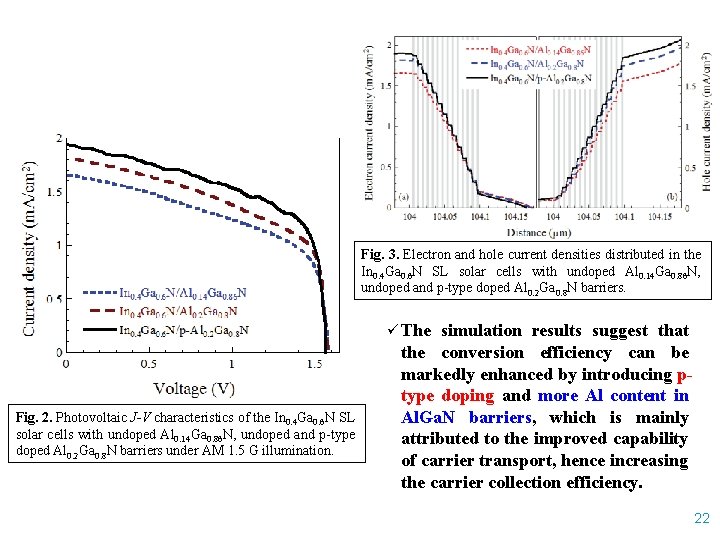
Fig. 3. Electron and hole current densities distributed in the In 0. 4 Ga 0. 6 N SL solar cells with undoped Al 0. 14 Ga 0. 86 N, undoped and p-type doped Al 0. 2 Ga 0. 8 N barriers. ü The Fig. 2. Photovoltaic J-V characteristics of the In 0. 4 Ga 0. 6 N SL solar cells with undoped Al 0. 14 Ga 0. 86 N, undoped and p-type doped Al 0. 2 Ga 0. 8 N barriers under AM 1. 5 G illumination. simulation results suggest that the conversion efficiency can be markedly enhanced by introducing ptype doping and more Al content in Al. Ga. N barriers, which is mainly attributed to the improved capability of carrier transport, hence increasing the carrier collection efficiency. 22
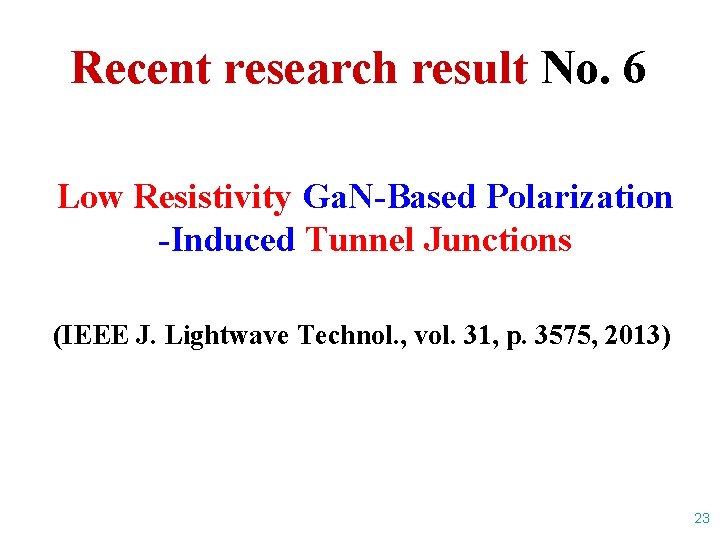
Recent research result No. 6 Low Resistivity Ga. N-Based Polarization -Induced Tunnel Junctions (IEEE J. Lightwave Technol. , vol. 31, p. 3575, 2013) 23
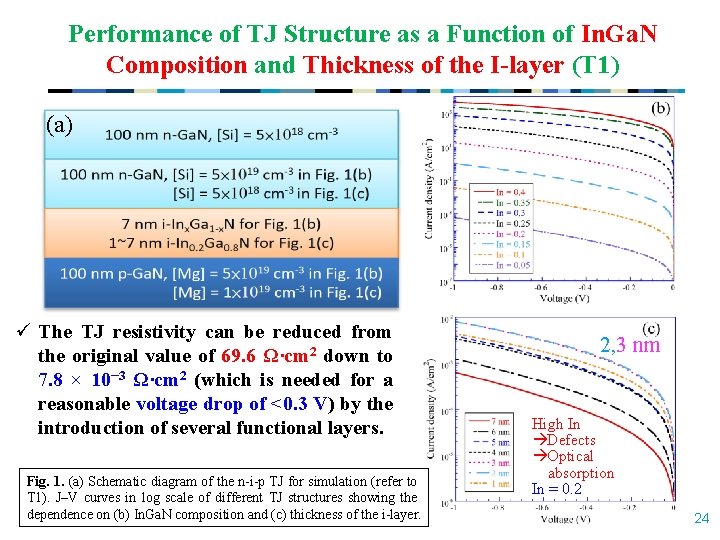
Performance of TJ Structure as a Function of In. Ga. N Composition and Thickness of the I-layer (T 1) (a) ü The TJ resistivity can be reduced from the original value of 69. 6 Ω·cm 2 down to 7. 8 × 10− 3 Ω·cm 2 (which is needed for a reasonable voltage drop of <0. 3 V) by the introduction of several functional layers. Fig. 1. (a) Schematic diagram of the n-i-p TJ for simulation (refer to T 1). J–V curves in log scale of different TJ structures showing the dependence on (b) In. Ga. N composition and (c) thickness of the i-layer. 2, 3 nm High In Defects Optical absorption In = 0. 2 24
![Dependence on Mg and Si Doping Densities T 2 a ü Firstly inserting two Dependence on [Mg] and [Si] Doping Densities (T 2) (a) ü Firstly, inserting two](https://slidetodoc.com/presentation_image_h2/d322b50bc80c8e7ce92697e89a1656ee/image-25.jpg)
Dependence on [Mg] and [Si] Doping Densities (T 2) (a) ü Firstly, inserting two thin (5 nm) highly-doped p- and n-Ga. N layers below and above the In. Ga. N layer to support more space charges. ü With 5 nm layers of p-Ga. N and n-Ga. N with [ND ] = 3 × 1019 cm− 3 and [NA] = 2 × 1019 cm− 3 (denoted as T 2), the resistivity of the TJ is reduced to 5 × 10− 2 Ω·cm 2. ü Further increasing the Mg doping level from 2 to 3 × 1019 cm− 3 does not increase the tunneling current appreciably, while increasing the Si doping changes the current density by almost an order of magnitude. Because of the large conduction band offset of the nitride materials, increasing the Si doping density is more effective than increasing the Mg doping density for improving the tunneling. Fig. 2. (a) Schematic diagram of the n-i-p TJ with the insertion of thin layers of different doping levels (refer to T 2). 25 (b) J–V curves in log scale.
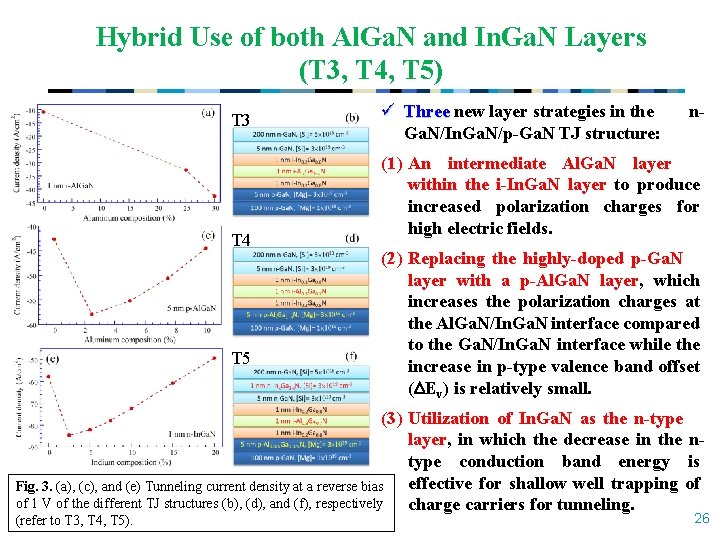
Hybrid Use of both Al. Ga. N and In. Ga. N Layers (T 3, T 4, T 5) T 3 T 4 T 5 ü Three new layer strategies in the Ga. N/In. Ga. N/p-Ga. N TJ structure: n- (1) An intermediate Al. Ga. N layer within the i-In. Ga. N layer to produce increased polarization charges for high electric fields. (2) Replacing the highly-doped p-Ga. N layer with a p-Al. Ga. N layer, which increases the polarization charges at the Al. Ga. N/In. Ga. N interface compared to the Ga. N/In. Ga. N interface while the increase in p-type valence band offset ( Ev) is relatively small. (3) Utilization of In. Ga. N as the n-type layer, in which the decrease in the ntype conduction band energy is effective for shallow well trapping of Fig. 3. (a), (c), and (e) Tunneling current density at a reverse bias of 1 V of the different TJ structures (b), (d), and (f), respectively charge carriers for tunneling. (refer to T 3, T 4, T 5). 26
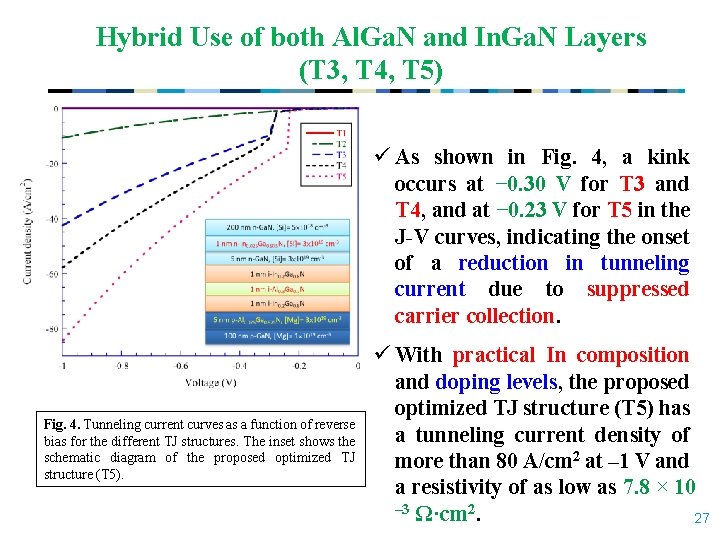
Hybrid Use of both Al. Ga. N and In. Ga. N Layers (T 3, T 4, T 5) ü As shown in Fig. 4, a kink occurs at − 0. 30 V for T 3 and T 4, and at − 0. 23 V for T 5 in the J-V curves, indicating the onset of a reduction in tunneling current due to suppressed carrier collection. Fig. 4. Tunneling current curves as a function of reverse bias for the different TJ structures. The inset shows the schematic diagram of the proposed optimized TJ structure (T 5). ü With practical In composition and doping levels, the proposed optimized TJ structure (T 5) has a tunneling current density of more than 80 A/cm 2 at – 1 V and a resistivity of as low as 7. 8 × 10 – 3 Ω·cm 2. 27
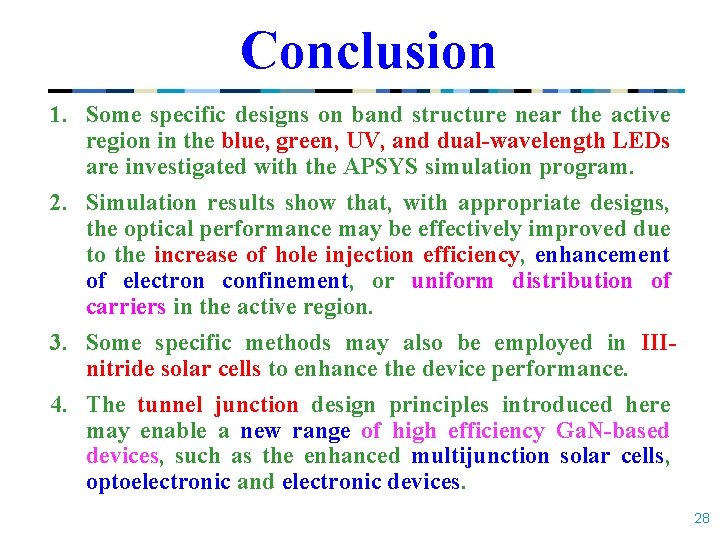
Conclusion 1. Some specific designs on band structure near the active region in the blue, green, UV, and dual-wavelength LEDs are investigated with the APSYS simulation program. 2. Simulation results show that, with appropriate designs, the optical performance may be effectively improved due to the increase of hole injection efficiency, enhancement of electron confinement, or uniform distribution of carriers in the active region. 3. Some specific methods may also be employed in IIInitride solar cells to enhance the device performance. 4. The tunnel junction design principles introduced here may enable a new range of high efficiency Ga. N-based devices, such as the enhanced multijunction solar cells, optoelectronic and electronic devices. 28
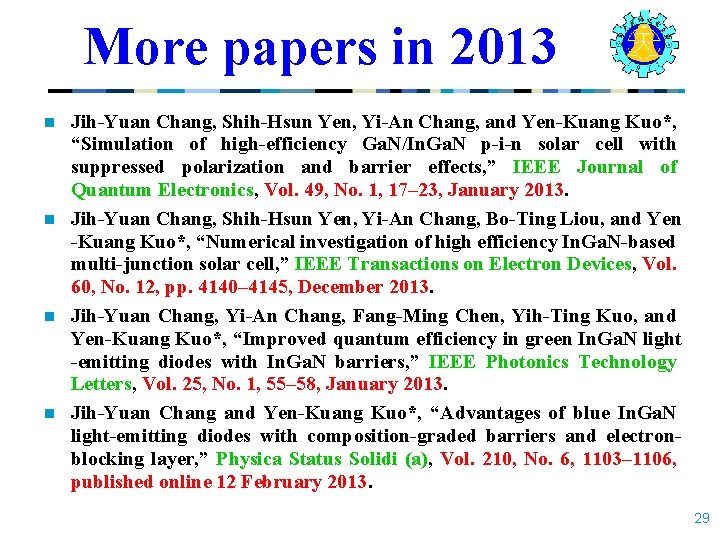
More papers in 2013 Jih-Yuan Chang, Shih-Hsun Yen, Yi-An Chang, and Yen-Kuang Kuo*, “Simulation of high-efficiency Ga. N/In. Ga. N p-i-n solar cell with suppressed polarization and barrier effects, ” IEEE Journal of Quantum Electronics, Vol. 49, No. 1, 17– 23, January 2013. n Jih-Yuan Chang, Shih-Hsun Yen, Yi-An Chang, Bo-Ting Liou, and Yen -Kuang Kuo*, “Numerical investigation of high efficiency In. Ga. N-based multi-junction solar cell, ” IEEE Transactions on Electron Devices, Vol. 60, No. 12, pp. 4140– 4145, December 2013. n Jih-Yuan Chang, Yi-An Chang, Fang-Ming Chen, Yih-Ting Kuo, and Yen-Kuang Kuo*, “Improved quantum efficiency in green In. Ga. N light -emitting diodes with In. Ga. N barriers, ” IEEE Photonics Technology Letters, Vol. 25, No. 1, 55– 58, January 2013. n Jih-Yuan Chang and Yen-Kuang Kuo*, “Advantages of blue In. Ga. N light-emitting diodes with composition-graded barriers and electronblocking layer, ” Physica Status Solidi (a), Vol. 210, No. 6, 1103– 1106, published online 12 February 2013. n 29
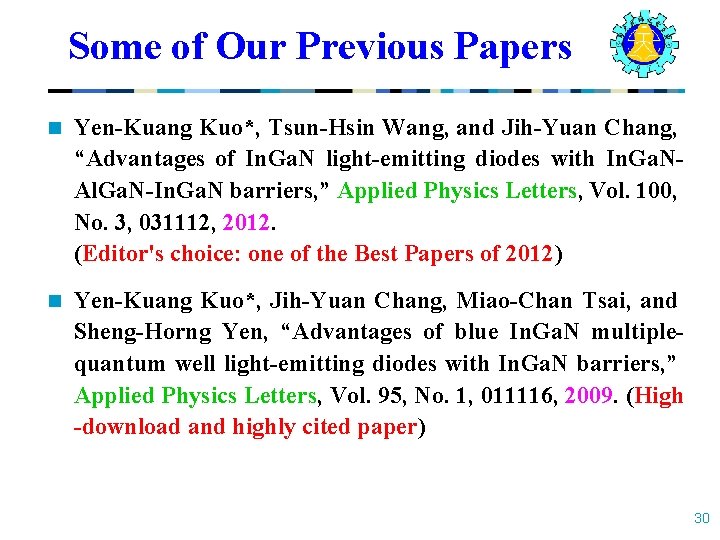
Some of Our Previous Papers n Yen-Kuang Kuo*, Tsun-Hsin Wang, and Jih-Yuan Chang, “Advantages of In. Ga. N light-emitting diodes with In. Ga. NAl. Ga. N-In. Ga. N barriers, ” Applied Physics Letters, Vol. 100, No. 3, 031112, 2012. (Editor's choice: one of the Best Papers of 2012) n Yen-Kuang Kuo*, Jih-Yuan Chang, Miao-Chan Tsai, and Sheng-Horng Yen, “Advantages of blue In. Ga. N multiplequantum well light-emitting diodes with In. Ga. N barriers, ” Applied Physics Letters, Vol. 95, No. 1, 011116, 2009. (High -download and highly cited paper) 30
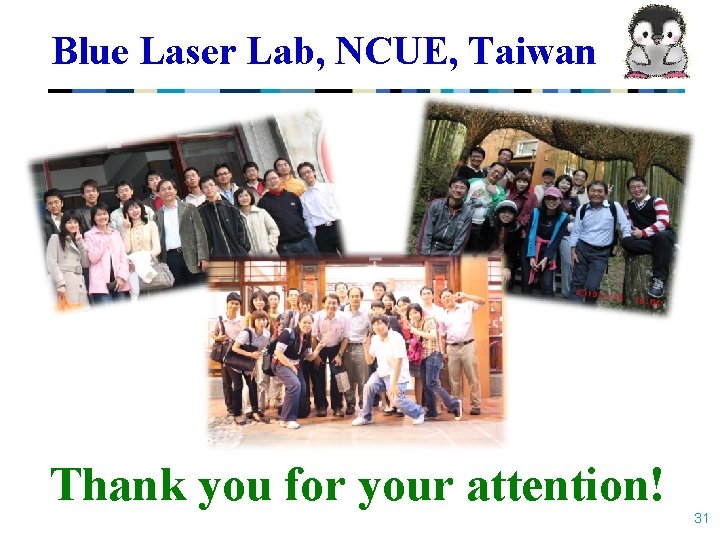
Blue Laser Lab, NCUE, Taiwan Thank you for your attention! 31
Ge diode
Solar cell wholesale
Solar energy is free. solar is inexhaustible
Diodes
Special purpose diodes
What are diodes made of
Zener diode exhibit
Zener diode equivalent circuit models
What are special purpose diodes
Solid state diode
Rectifier advantages and disadvantages
Zener diode application
Diode symbol
Practical diode model
Simbol za tranzistor
Introduction to diodes
Circuit analysis with diodes
Special purpose diodes
Disadvantages of optical fibre
Axons of ganglion cells
Pituitary and optic chiasm
Hypophysis
Fiber optic hub switch
Pie in the sky lesion
Slit lamp illumination
Uniaxial interference figure
Disadvantages of fiber optics
Atrophy
Optic graphic organizer
Magnetic optical current transformer
Microsens distributor
Microscopul optic