EAT 347 PART II Case Example of Chemodynamics
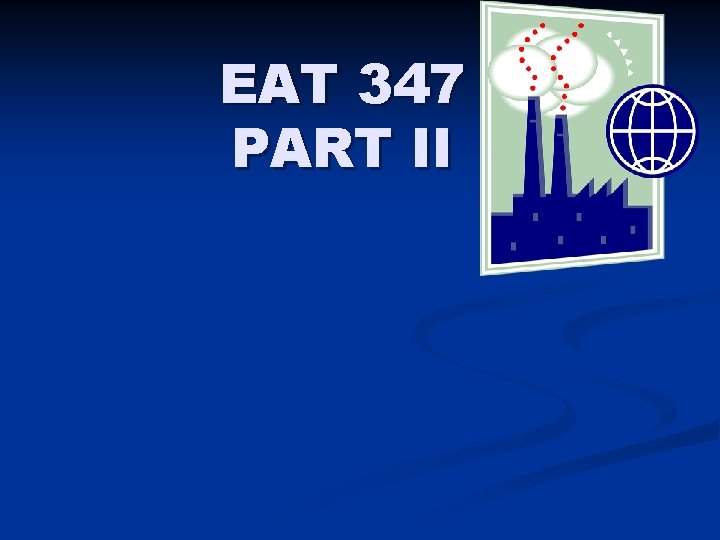
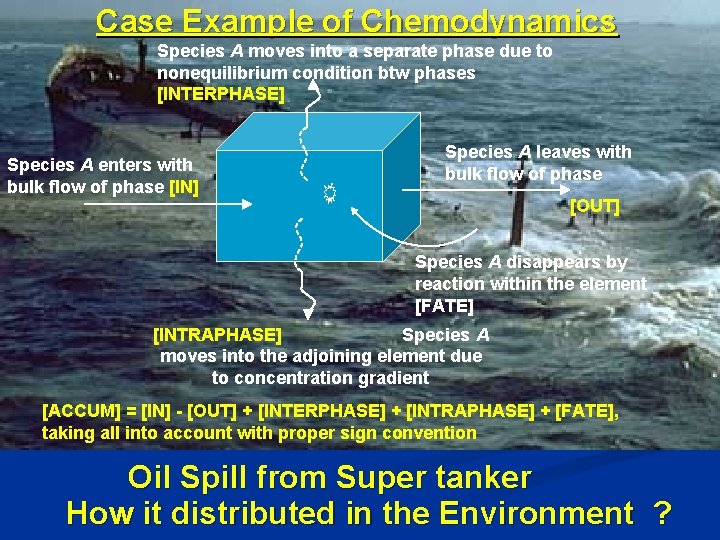
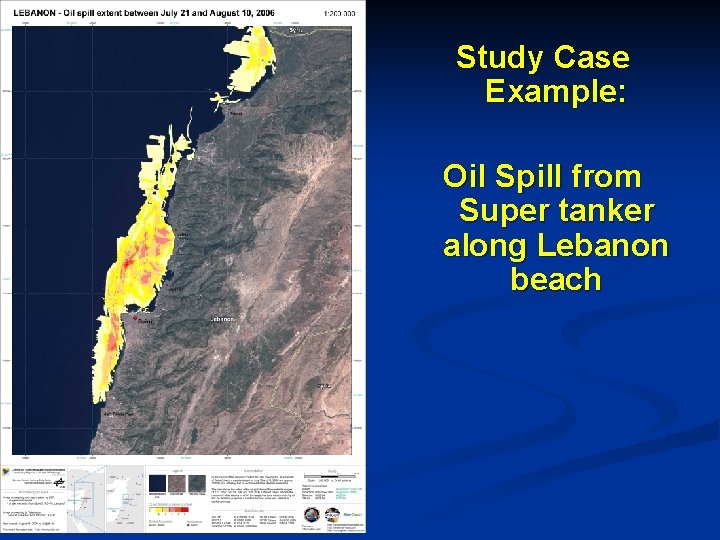
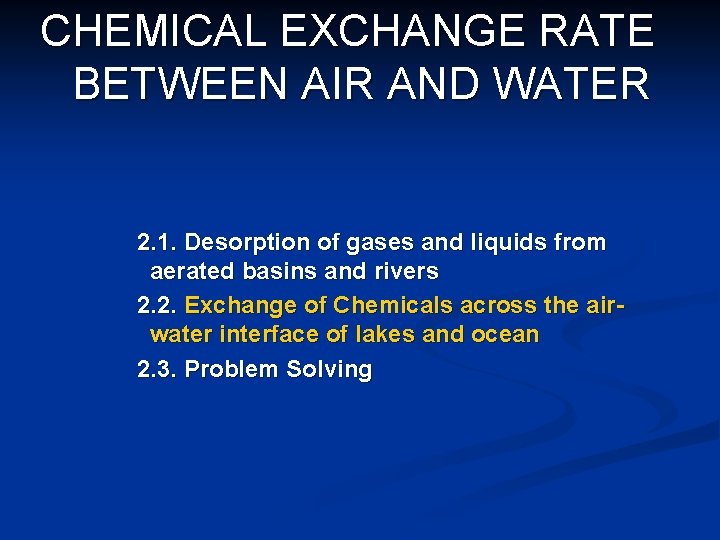
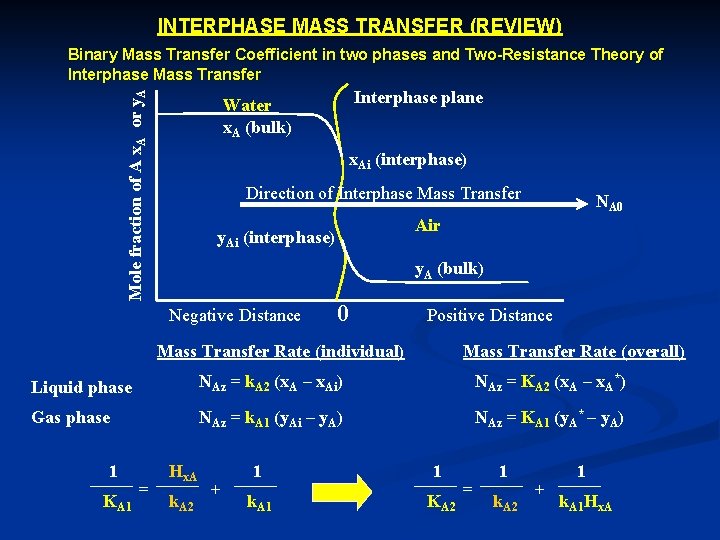
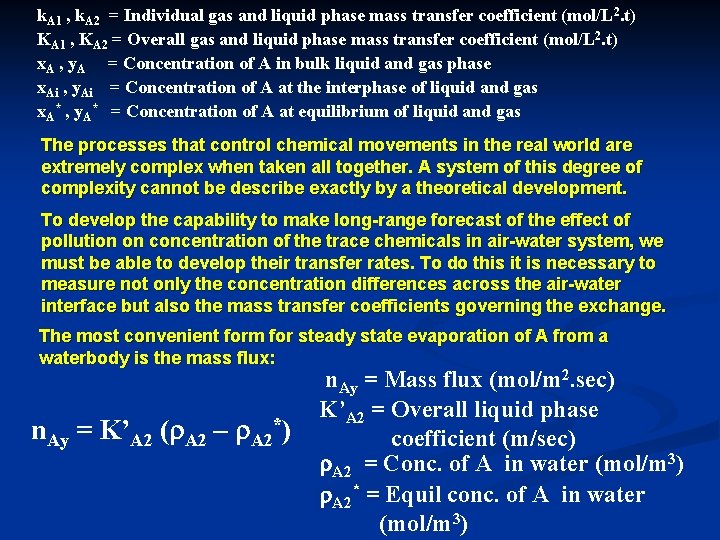
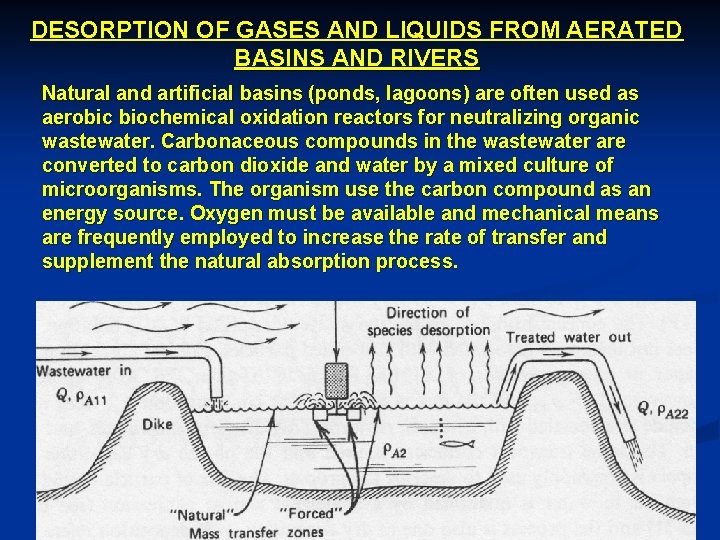
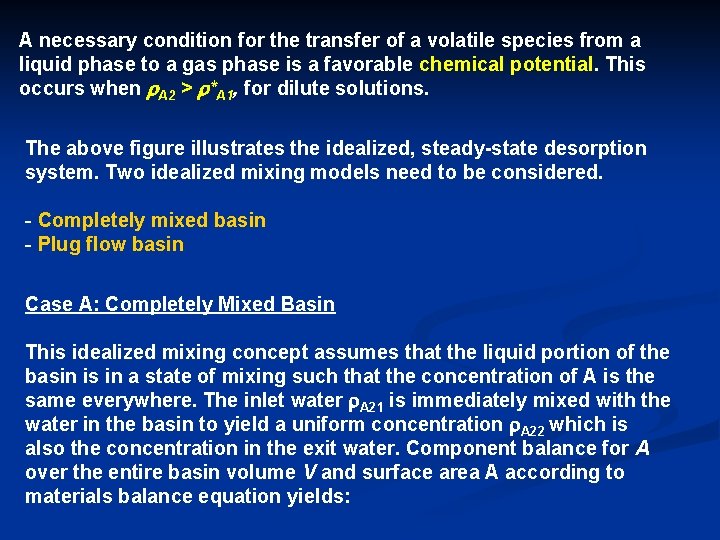
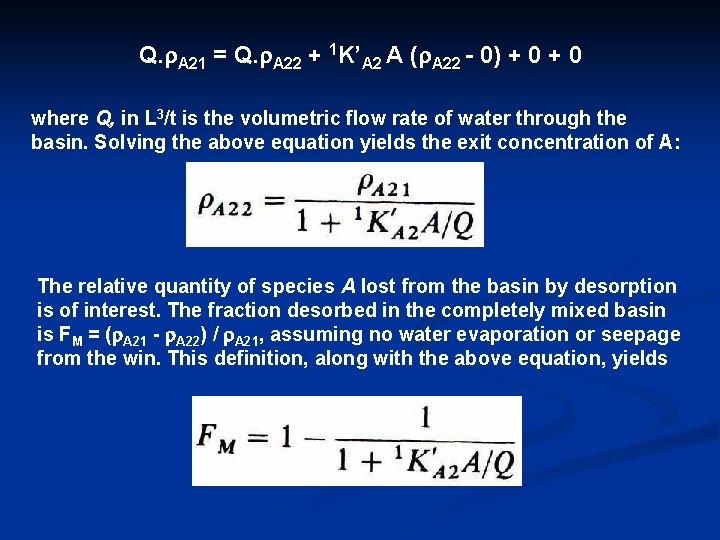
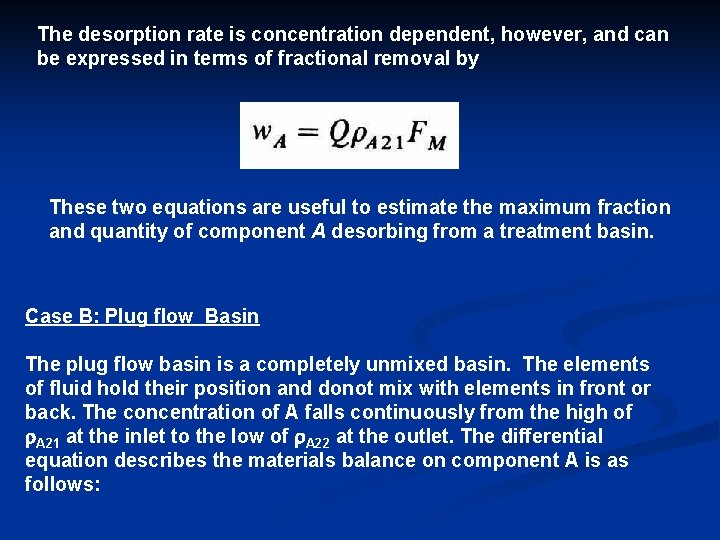
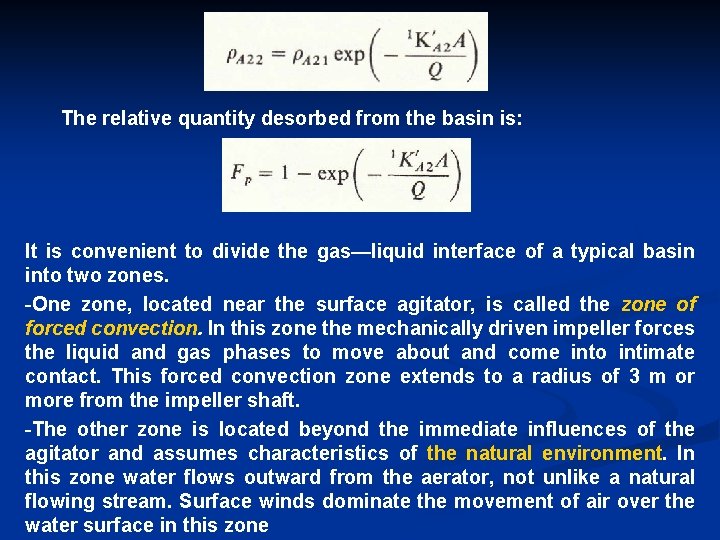
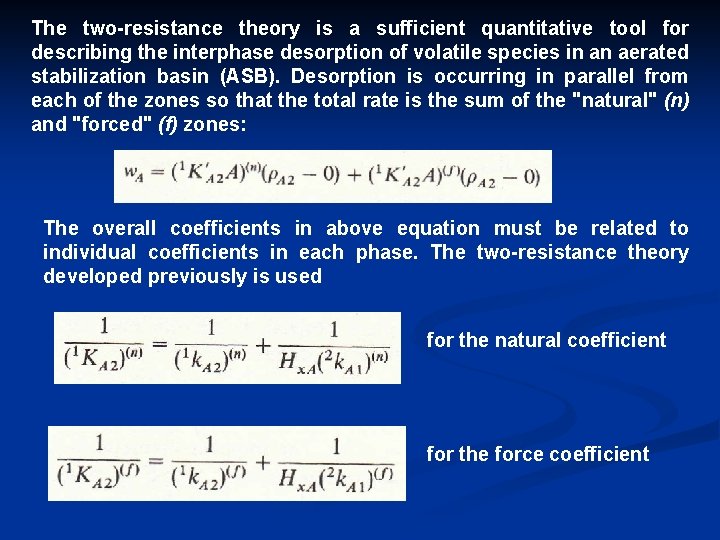
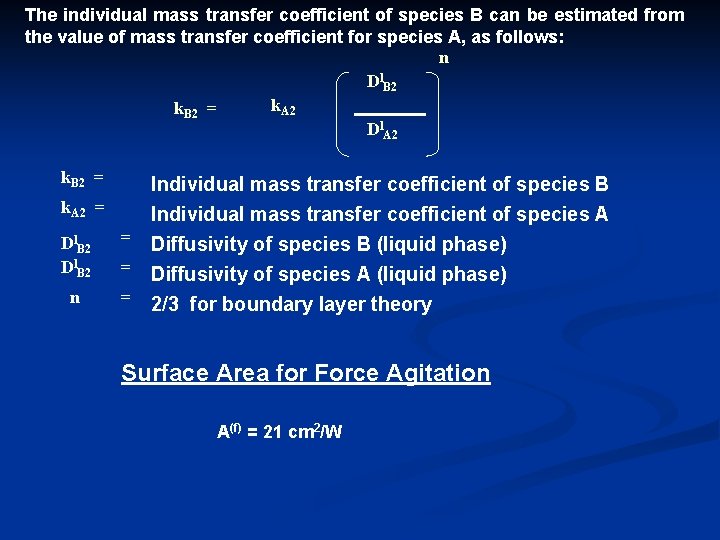
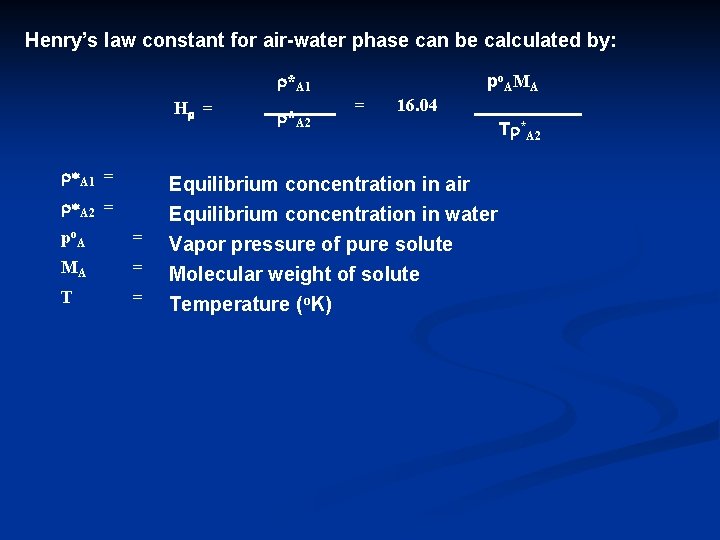
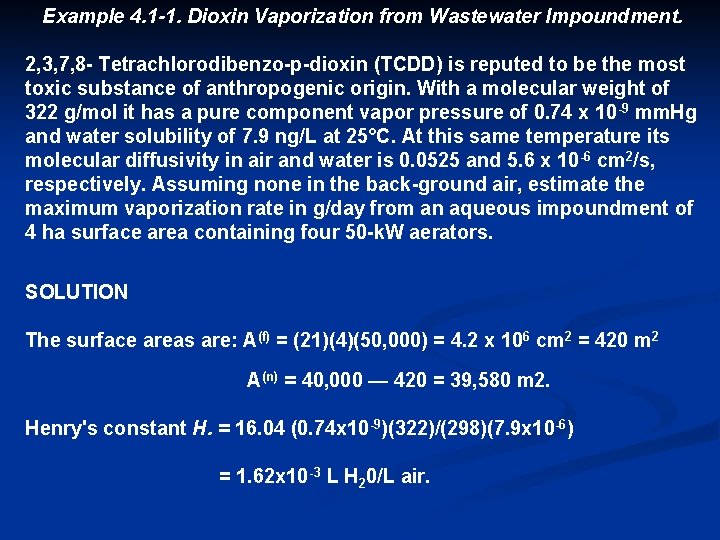
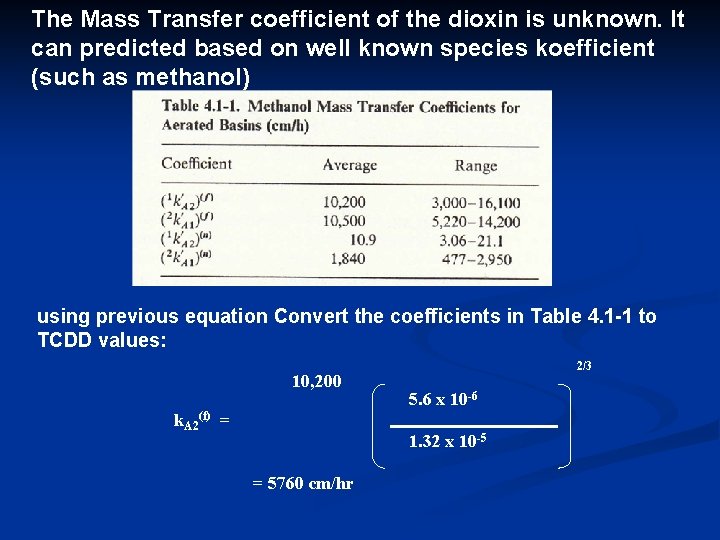
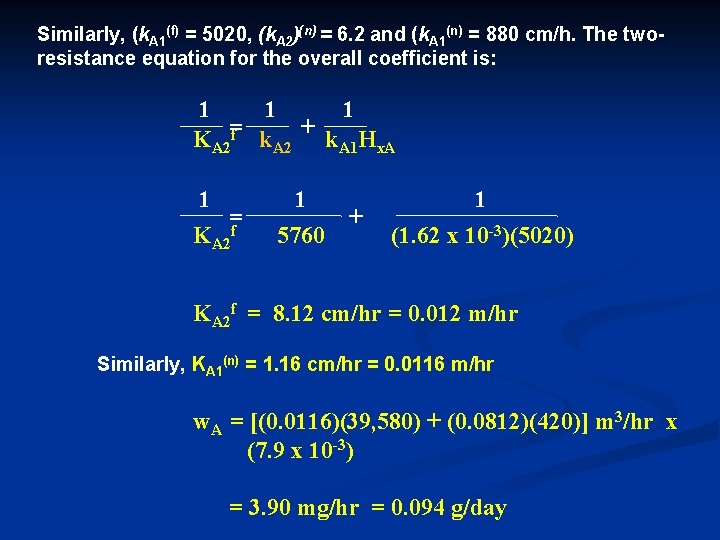
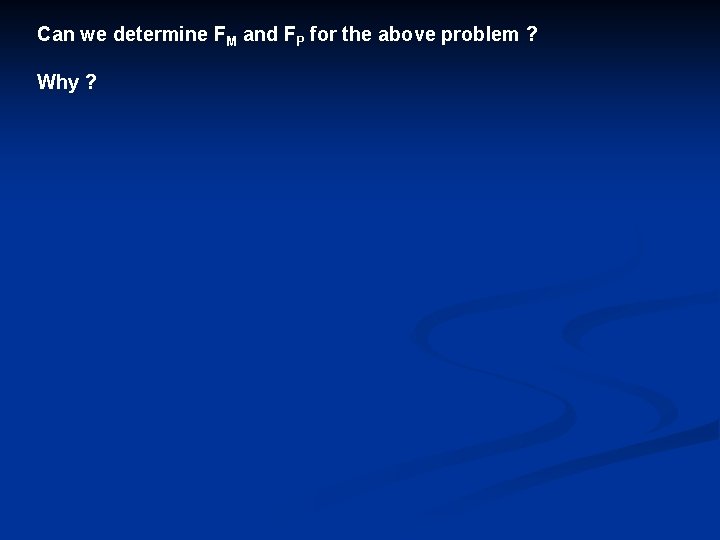
- Slides: 18
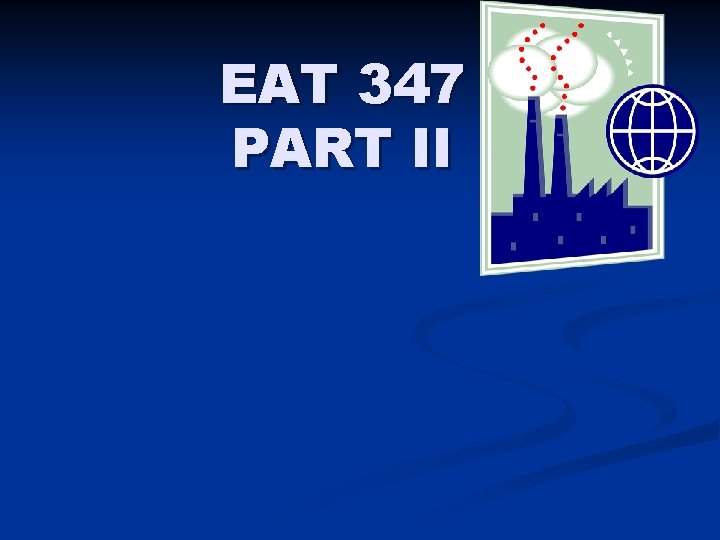
EAT 347 PART II
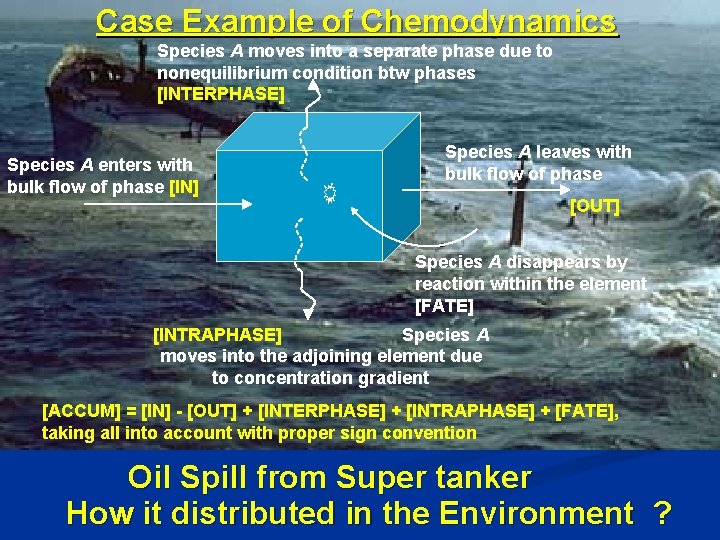
Case Example of Chemodynamics Species A moves into a separate phase due to nonequilibrium condition btw phases [INTERPHASE] Species A enters with bulk flow of phase [IN] Species A leaves with bulk flow of phase [OUT] Species A disappears by reaction within the element [FATE] [INTRAPHASE] Species A moves into the adjoining element due to concentration gradient [ACCUM] = [IN] [OUT] + [INTERPHASE] + [INTRAPHASE] + [FATE], taking all into account with proper sign convention Oil Spill from Super tanker How it distributed in the Environment ?
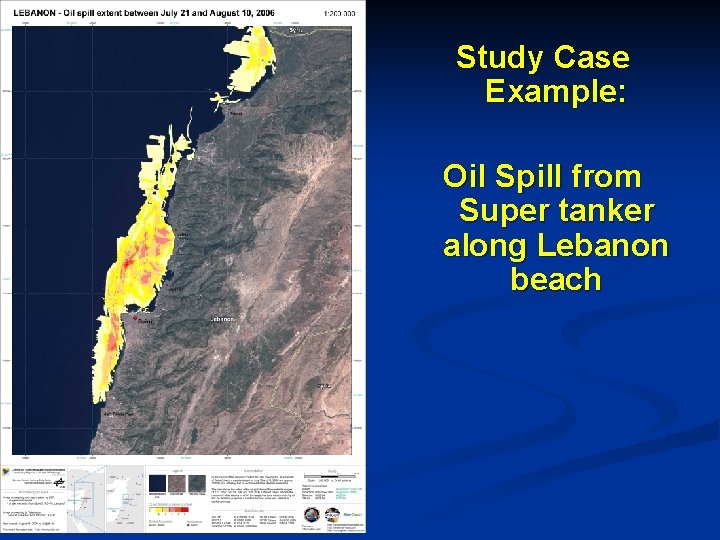
Study Case Example: Oil Spill from Super tanker along Lebanon beach
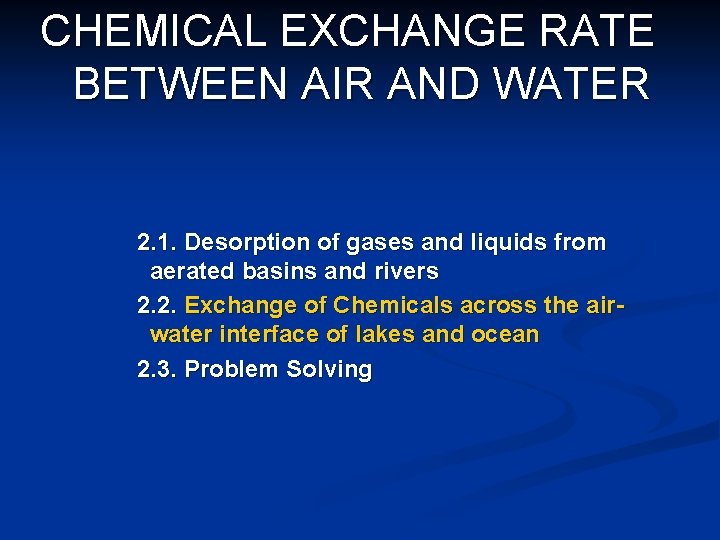
CHEMICAL EXCHANGE RATE BETWEEN AIR AND WATER 2. 1. Desorption of gases and liquids from aerated basins and rivers 2. 2. Exchange of Chemicals across the air water interface of lakes and ocean 2. 3. Problem Solving
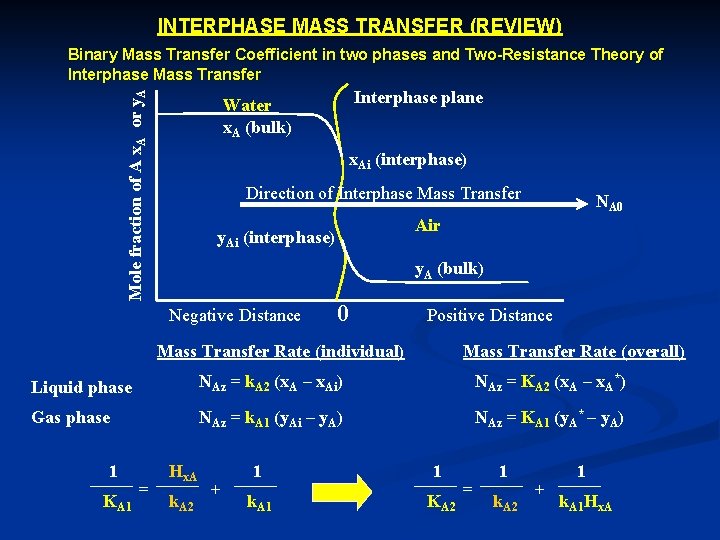
INTERPHASE MASS TRANSFER (REVIEW) Mole fraction of A x. A or y. A Binary Mass Transfer Coefficient in two phases and Two Resistance Theory of Interphase Mass Transfer Interphase plane Water x. A (bulk) x. Ai (interphase) Direction of Interphase Mass Transfer NA 0 Air y. Ai (interphase) y. A (bulk) Negative Distance 0 Positive Distance Mass Transfer Rate (individual) Mass Transfer Rate (overall) Liquid phase NAz = k. A 2 (x. A – x. Ai) NAz = KA 2 (x. A – x. A*) Gas phase NAz = k. A 1 (y. Ai – y. A) NAz = KA 1 (y. A* – y. A) 1 KA 1 = Hx. A k. A 2 + 1 1 k. A 1 KA 2 = 1 k. A 2 + 1 k. A 1 Hx. A
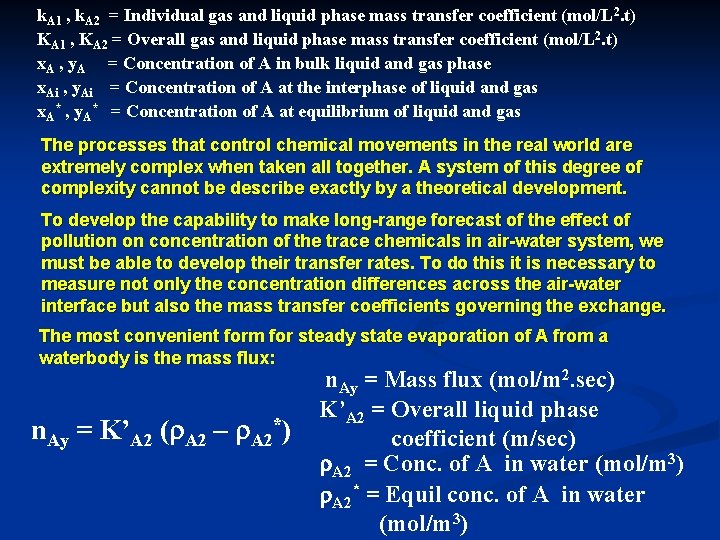
k. A 1 , k. A 2 = Individual gas and liquid phase mass transfer coefficient (mol/L 2. t) KA 1 , KA 2 = Overall gas and liquid phase mass transfer coefficient (mol/L 2. t) x. A , y. A = Concentration of A in bulk liquid and gas phase x. Ai , y. Ai = Concentration of A at the interphase of liquid and gas x. A* , y. A* = Concentration of A at equilibrium of liquid and gas The processes that control chemical movements in the real world are extremely complex when taken all together. A system of this degree of complexity cannot be describe exactly by a theoretical development. To develop the capability to make long range forecast of the effect of pollution on concentration of the trace chemicals in air water system, we must be able to develop their transfer rates. To do this it is necessary to measure not only the concentration differences across the air water interface but also the mass transfer coefficients governing the exchange. The most convenient form for steady state evaporation of A from a waterbody is the mass flux: n. Ay = K’A 2 (r. A 2 – r. A 2*) n. Ay = Mass flux (mol/m 2. sec) K’A 2 = Overall liquid phase coefficient (m/sec) r. A 2 = Conc. of A in water (mol/m 3) r. A 2* = Equil conc. of A in water (mol/m 3)
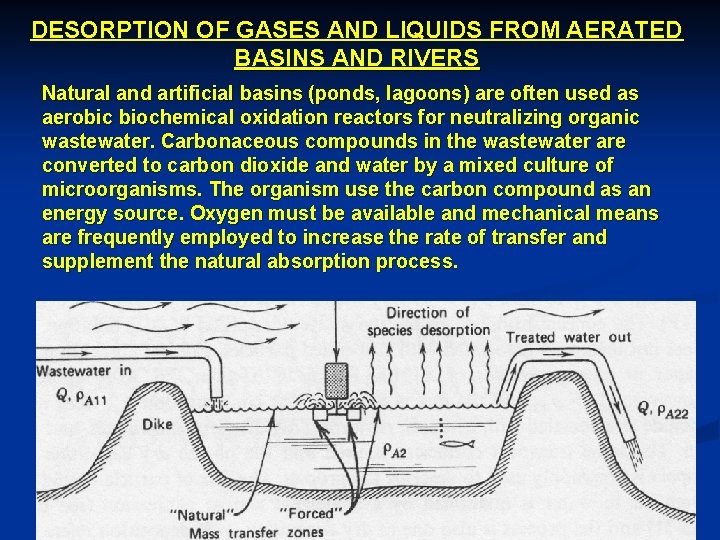
DESORPTION OF GASES AND LIQUIDS FROM AERATED BASINS AND RIVERS Natural and artificial basins (ponds, lagoons) are often used as aerobic biochemical oxidation reactors for neutralizing organic wastewater. Carbonaceous compounds in the wastewater are converted to carbon dioxide and water by a mixed culture of microorganisms. The organism use the carbon compound as an energy source. Oxygen must be available and mechanical means are frequently employed to increase the rate of transfer and supplement the natural absorption process.
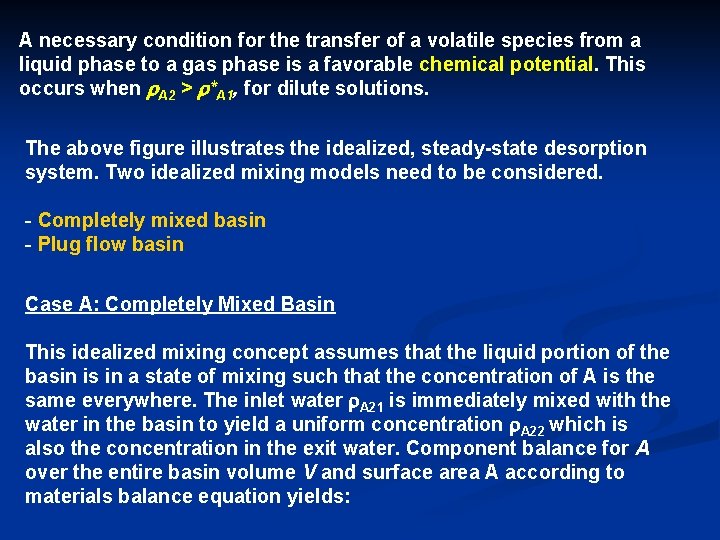
A necessary condition for the transfer of a volatile species from a liquid phase to a gas phase is a favorable chemical potential. This occurs when r. A 2 > r*A 1, for dilute solutions. The above figure illustrates the idealized, steady state desorption system. Two idealized mixing models need to be considered. - Completely mixed basin - Plug flow basin Case A: Completely Mixed Basin This idealized mixing concept assumes that the liquid portion of the basin is in a state of mixing such that the concentration of A is the same everywhere. The inlet water r. A 21 is immediately mixed with the water in the basin to yield a uniform concentration r. A 22 which is also the concentration in the exit water. Component balance for A over the entire basin volume V and surface area A according to materials balance equation yields:
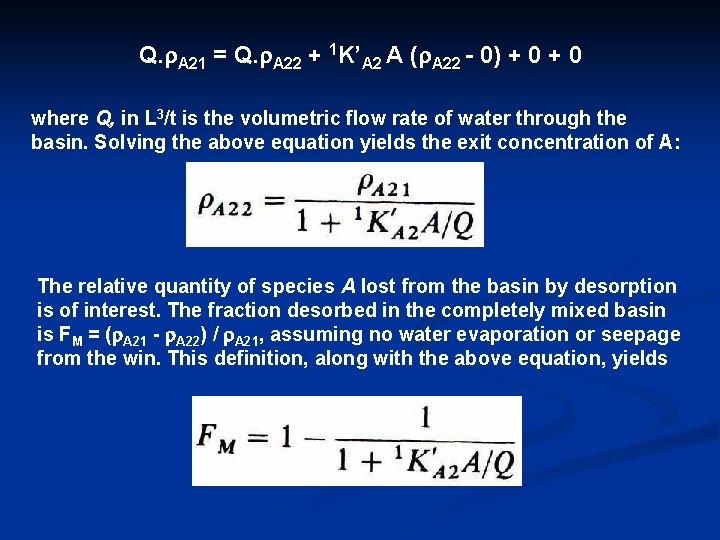
Q. r. A 21 = Q. r. A 22 + 1 K’A 2 A (r. A 22 0) + 0 where Q, in L 3/t is the volumetric flow rate of water through the basin. Solving the above equation yields the exit concentration of A: The relative quantity of species A lost from the basin by desorption is of interest. The fraction desorbed in the completely mixed basin is FM = (r. A 21 r. A 22) / r. A 21, assuming no water evaporation or seepage from the win. This definition, along with the above equation, yields
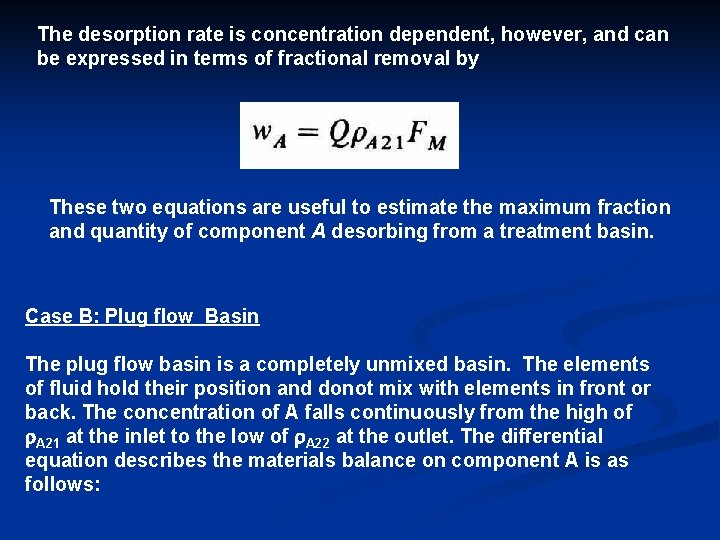
The desorption rate is concentration dependent, however, and can be expressed in terms of fractional removal by These two equations are useful to estimate the maximum fraction and quantity of component A desorbing from a treatment basin. Case B: Plug flow Basin The plug flow basin is a completely unmixed basin. The elements of fluid hold their position and donot mix with elements in front or back. The concentration of A falls continuously from the high of r. A 21 at the inlet to the low of r. A 22 at the outlet. The differential equation describes the materials balance on component A is as follows:
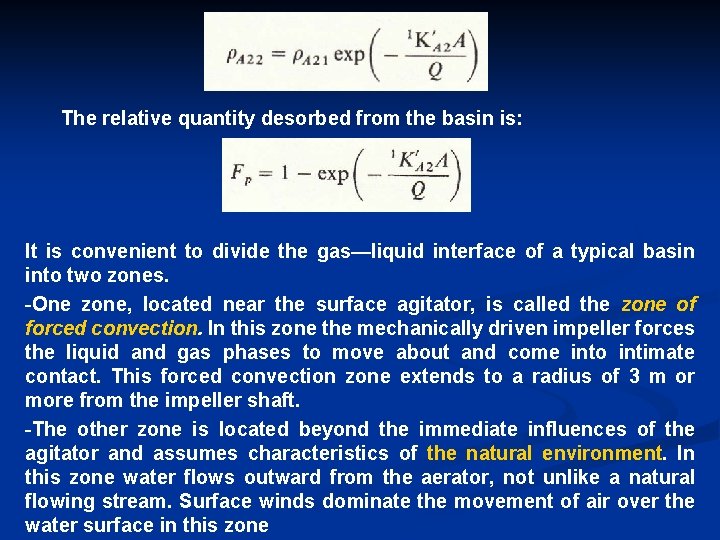
The relative quantity desorbed from the basin is: It is convenient to divide the gas—liquid interface of a typical basin into two zones. -One zone, located near the surface agitator, is called the zone of forced convection. In this zone the mechanically driven impeller forces the liquid and gas phases to move about and come into intimate contact. This forced convection zone extends to a radius of 3 m or more from the impeller shaft. -The other zone is located beyond the immediate influences of the agitator and assumes characteristics of the natural environment. In this zone water flows outward from the aerator, not unlike a natural flowing stream. Surface winds dominate the movement of air over the water surface in this zone
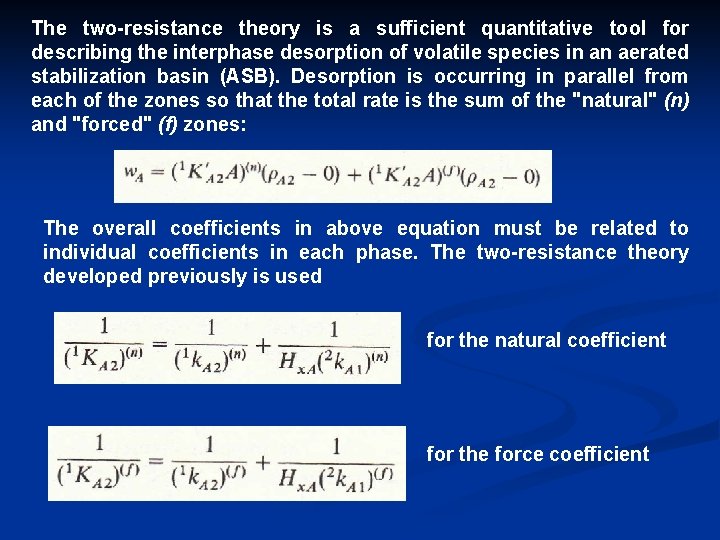
The two resistance theory is a sufficient quantitative tool for describing the interphase desorption of volatile species in an aerated stabilization basin (ASB). Desorption is occurring in parallel from each of the zones so that the total rate is the sum of the "natural" (n) and "forced" (f) zones: The overall coefficients in above equation must be related to individual coefficients in each phase. The two resistance theory developed previously is used for the natural coefficient for the force coefficient
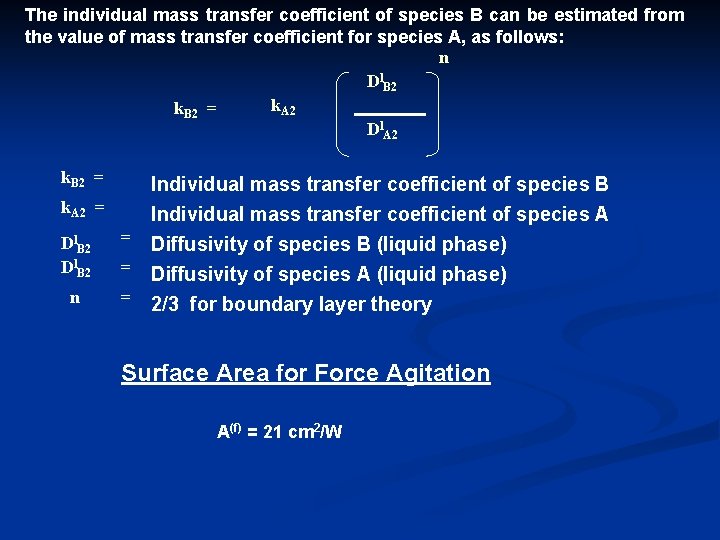
The individual mass transfer coefficient of species B can be estimated from the value of mass transfer coefficient for species A, as follows: n Dl. B 2 k. A 2 k. B 2 = Dl. A 2 k. B 2 = Individual mass transfer coefficient of species B k. A 2 = Individual mass transfer coefficient of species A = Diffusivity of species B (liquid phase) Dl. B 2 n = Diffusivity of species A (liquid phase) = 2/3 for boundary layer theory Surface Area for Force Agitation A(f) = 21 cm 2/W
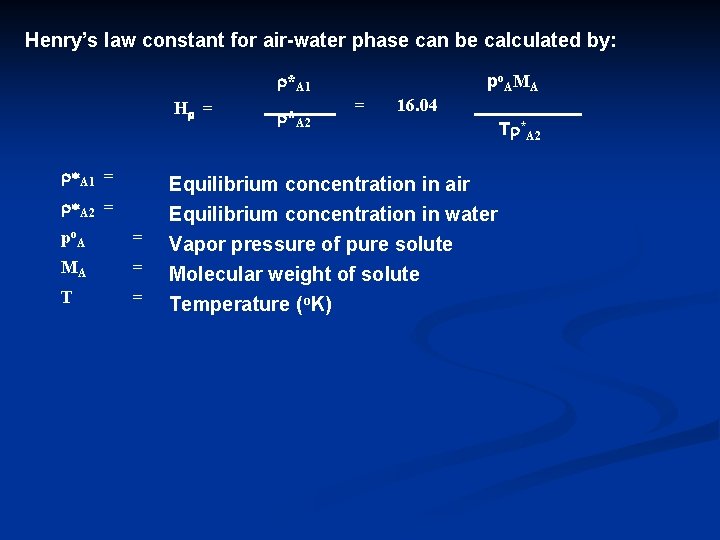
Henry’s law constant for air water phase can be calculated by: r*A 1 Hr = r*A 1 = r*A 2 = po A = MA = T = r*A 2 po A MA = 16. 04 Equilibrium concentration in air Equilibrium concentration in water Vapor pressure of pure solute Molecular weight of solute Temperature (o. K) Tr*A 2
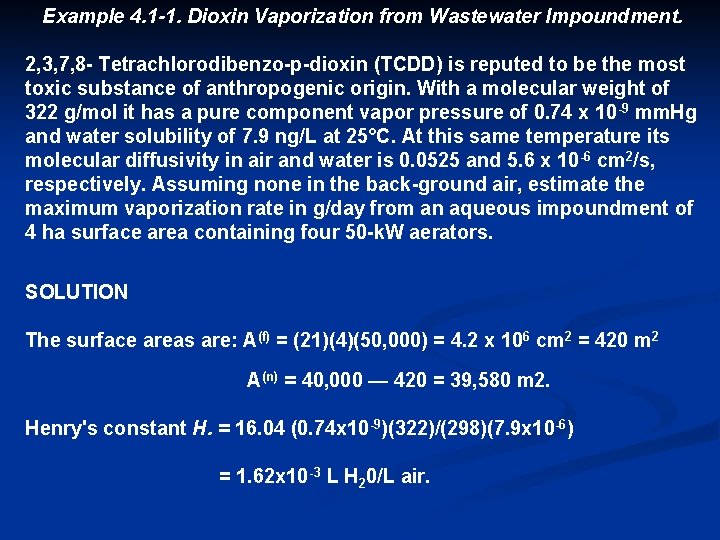
Example 4. 1 -1. Dioxin Vaporization from Wastewater Impoundment. 2, 3, 7, 8 Tetrachlorodibenzo p dioxin (TCDD) is reputed to be the most toxic substance of anthropogenic origin. With a molecular weight of 322 g/mol it has a pure component vapor pressure of 0. 74 x 10 9 mm. Hg and water solubility of 7. 9 ng/L at 25°C. At this same temperature its molecular diffusivity in air and water is 0. 0525 and 5. 6 x 10 6 cm 2/s, respectively. Assuming none in the back ground air, estimate the maximum vaporization rate in g/day from an aqueous impoundment of 4 ha surface area containing four 50 k. W aerators. SOLUTION The surface areas are: A(f) = (21)(4)(50, 000) = 4. 2 x 106 cm 2 = 420 m 2 A(n) = 40, 000 — 420 = 39, 580 m 2. Henry's constant H. = 16. 04 (0. 74 x 10 9)(322)/(298)(7. 9 x 10 6) = 1. 62 x 10 3 L H 20/L air.
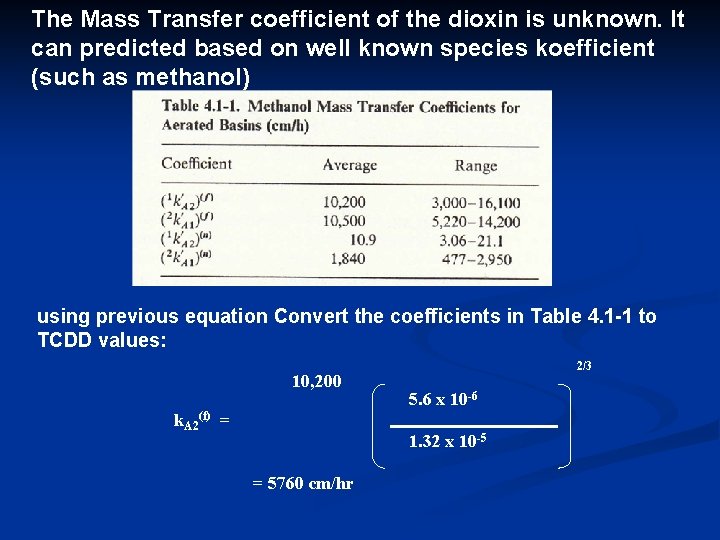
The Mass Transfer coefficient of the dioxin is unknown. It can predicted based on well known species koefficient (such as methanol) using previous equation Convert the coefficients in Table 4. 1 1 to TCDD values: 10, 200 k. A 2(f) = 2/3 5. 6 x 10 -6 1. 32 x 10 -5 = 5760 cm/hr
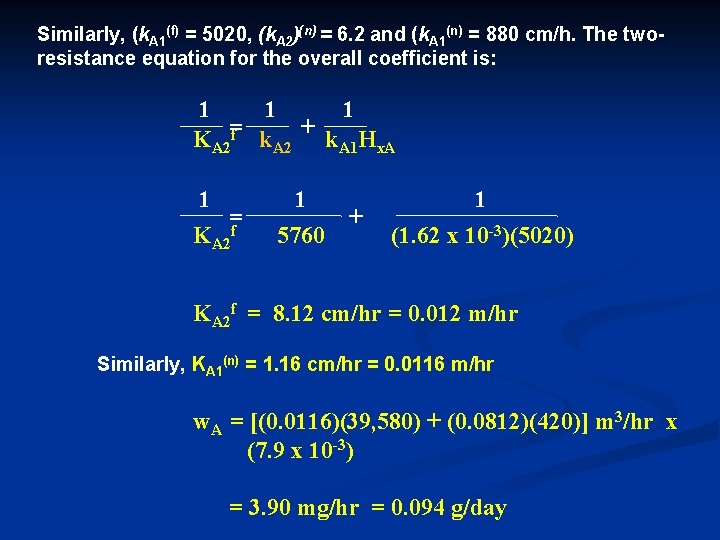
Similarly, (k. A 1(f) = 5020, (k. A 2)(n) = 6. 2 and (k. A 1(n) = 880 cm/h. The two resistance equation for the overall coefficient is: 1 1 1 KA 2=f k. A 2 + k. A 1 Hx. A 1 =f KA 2 1 5760 + 1 (1. 62 x 10 -3)(5020) KA 2 f = 8. 12 cm/hr = 0. 012 m/hr Similarly, KA 1(n) = 1. 16 cm/hr = 0. 0116 m/hr w. A = [(0. 0116)(39, 580) + (0. 0812)(420)] m 3/hr x (7. 9 x 10 -3) = 3. 90 mg/hr = 0. 094 g/day
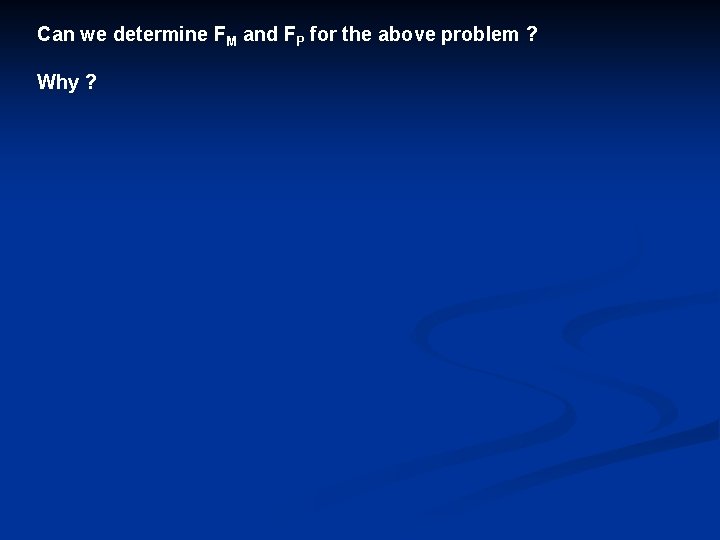
Can we determine FM and FP for the above problem ? Why ?
Eat 347
I would rather eat potatoes than to eat rice answer
I eat you eat he eats
People buy me to eat
Best case worst case average case
Opwekking 723 tekst
Plato 427 347 bc
Plato (427-347 sm)
Rtca do-311a
Cs 347
Cs 347
Platon (427-347 a.c)
Platon (427-347 a.c)
Plato (427-347 sm)
Plato 427 347 bc
347 000 in scientific notation
Scientific notation rules
Plato 427 347 bc
Alena ocilkova