Attenuation of photons in matter Lecture 4 Photon
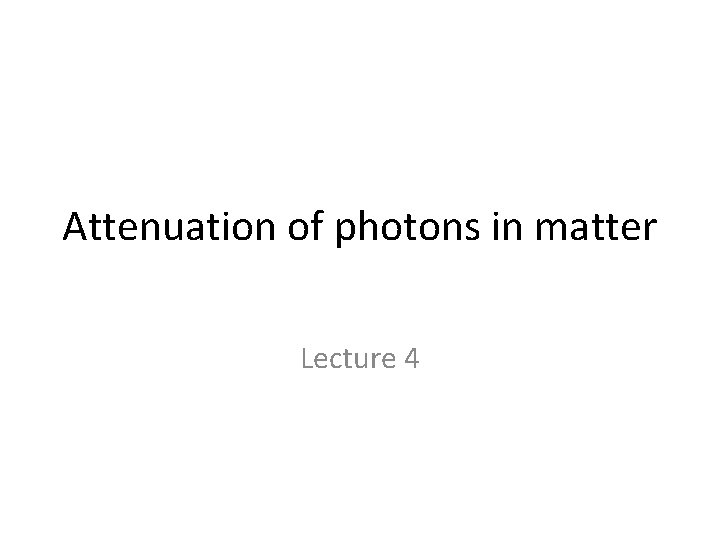
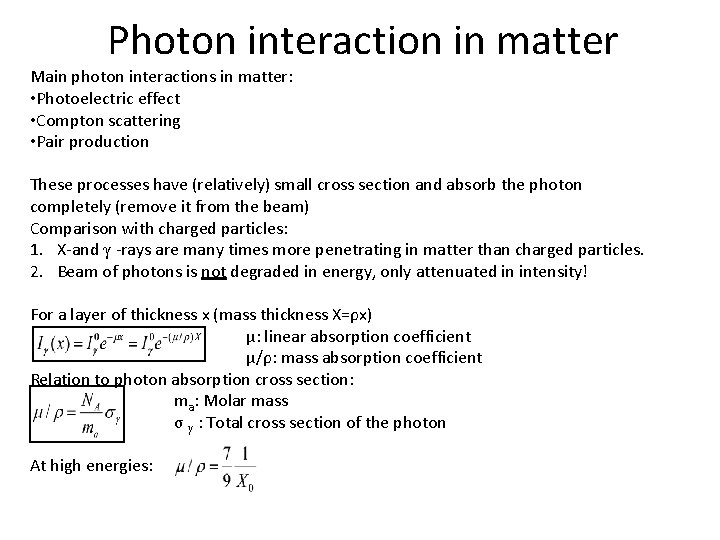
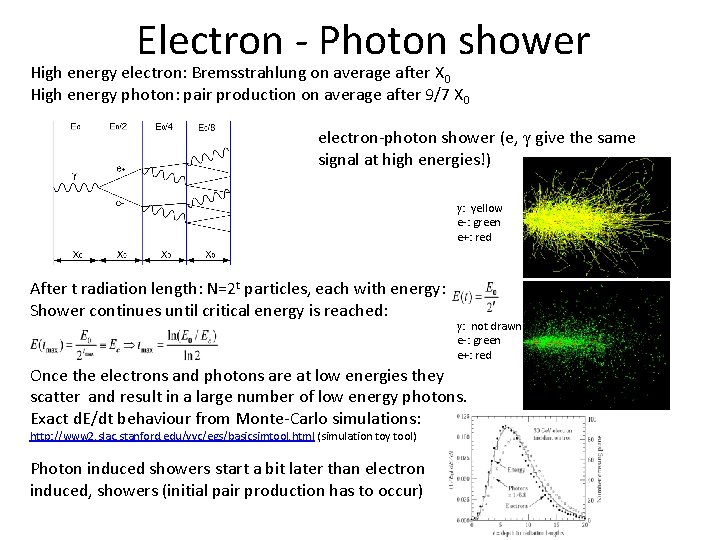
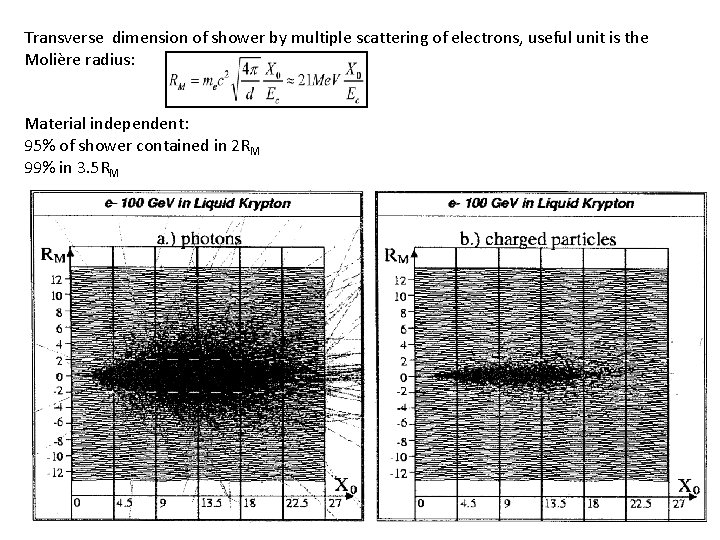
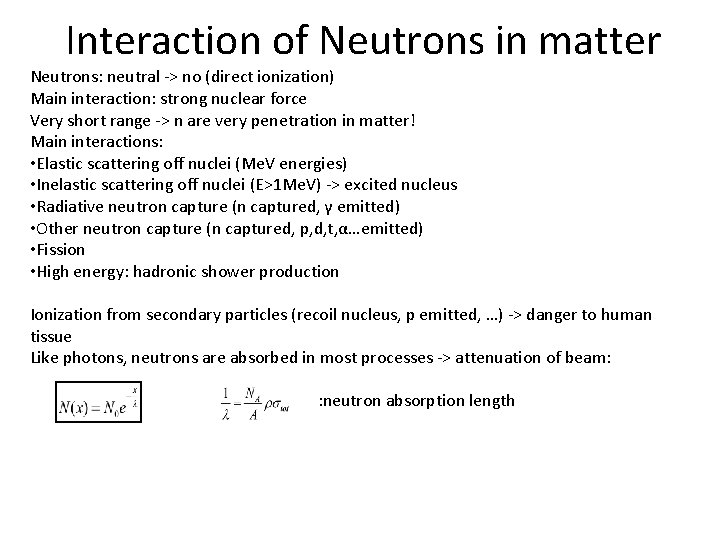
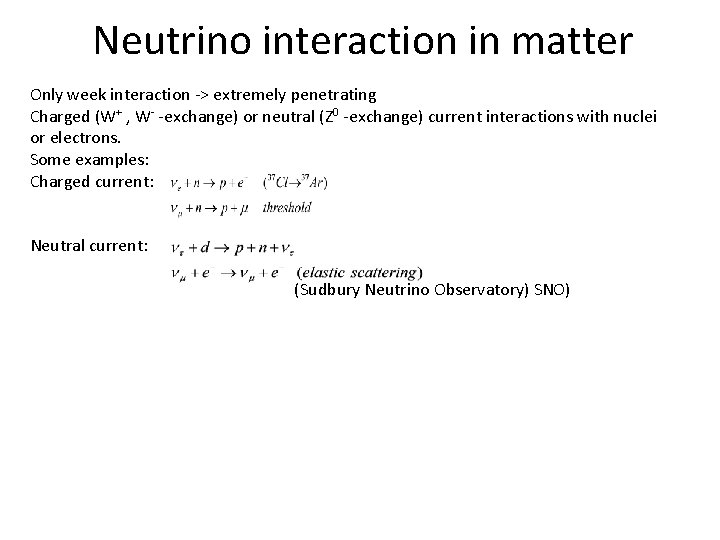
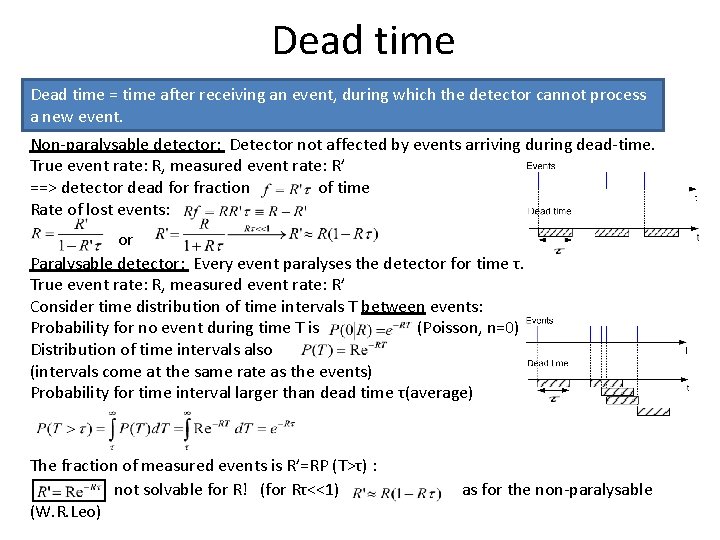
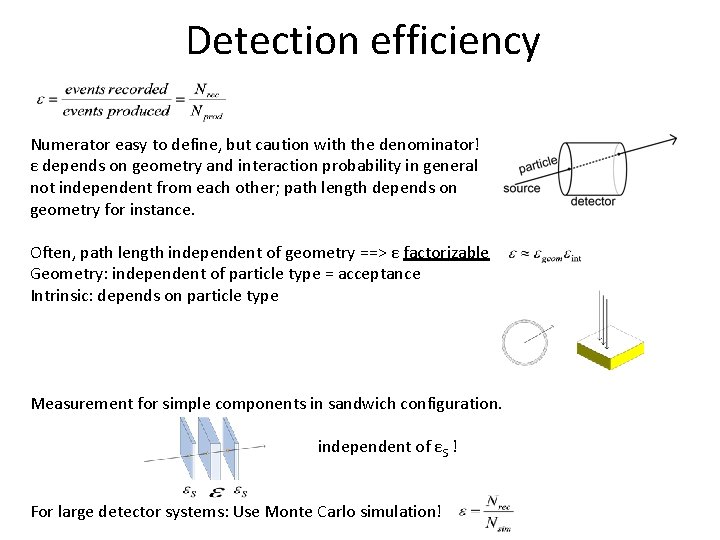
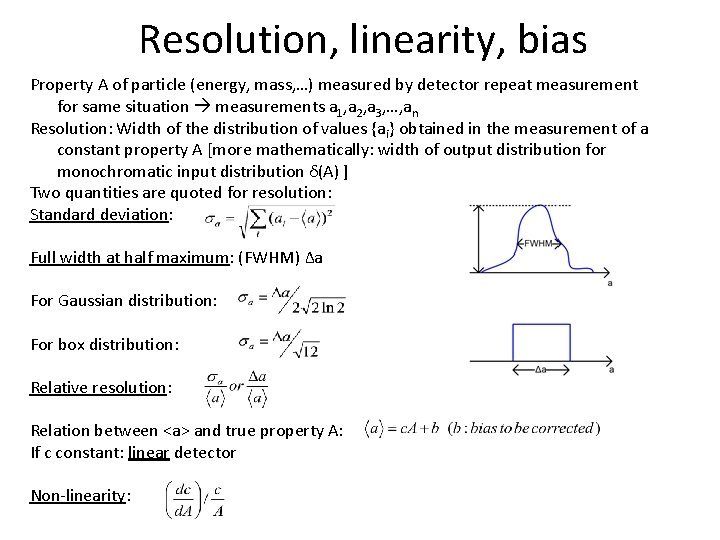
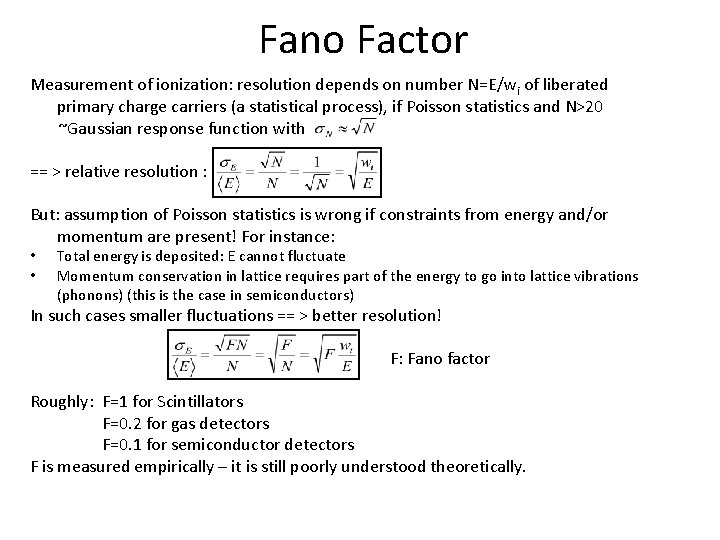
- Slides: 10
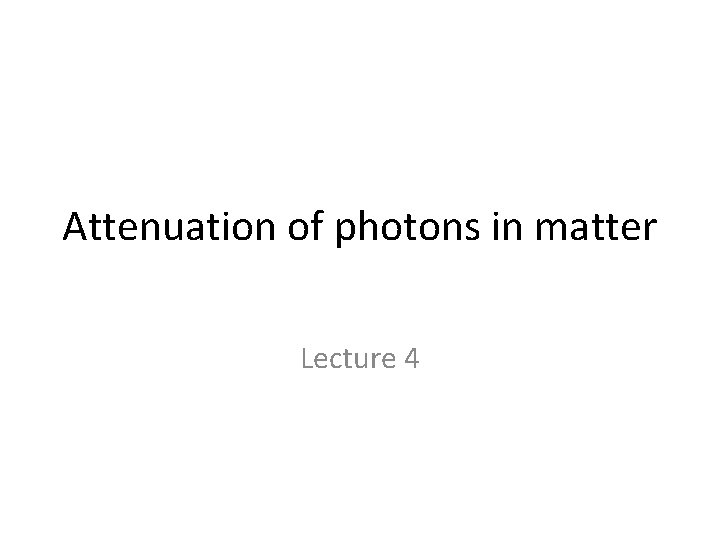
Attenuation of photons in matter Lecture 4
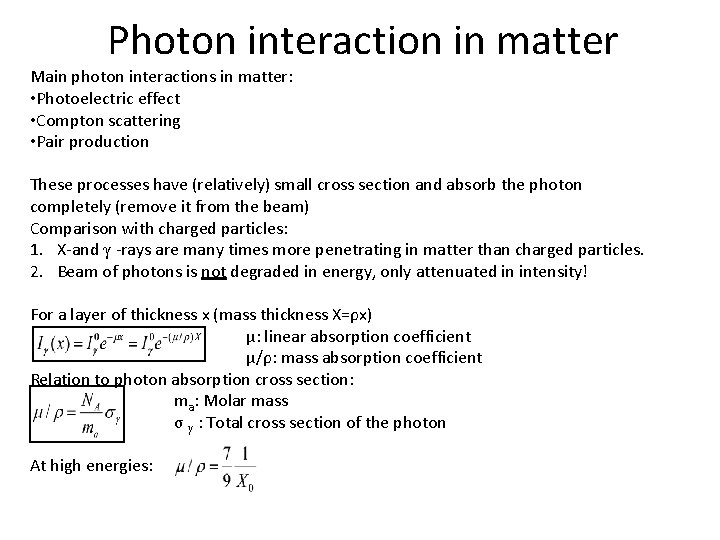
Photon interaction in matter Main photon interactions in matter: • Photoelectric effect • Compton scattering • Pair production These processes have (relatively) small cross section and absorb the photon completely (remove it from the beam) Comparison with charged particles: 1. X-and γ -rays are many times more penetrating in matter than charged particles. 2. Beam of photons is not degraded in energy, only attenuated in intensity! For a layer of thickness x (mass thickness X=ρx) μ: linear absorption coefficient μ/ρ: mass absorption coefficient Relation to photon absorption cross section: ma: Molar mass σ γ : Total cross section of the photon At high energies:
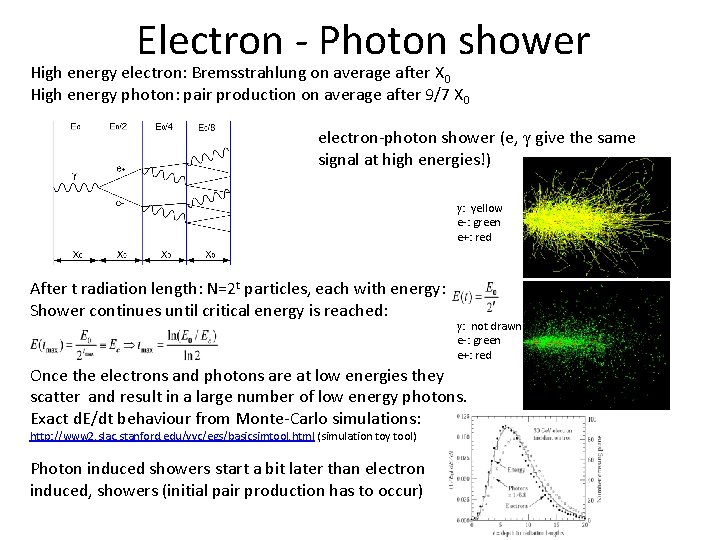
Electron - Photon shower High energy electron: Bremsstrahlung on average after X 0 High energy photon: pair production on average after 9/7 X 0 electron-photon shower (e, γ give the same signal at high energies!) γ: yellow e-: green e+: red After t radiation length: N=2 t particles, each with energy: Shower continues until critical energy is reached: γ: not drawn e-: green e+: red Once the electrons and photons are at low energies they scatter and result in a large number of low energy photons. Exact d. E/dt behaviour from Monte-Carlo simulations: http: //www 2. slac. stanford. edu/vvc/egs/basicsimtool. html (simulation toy tool) Photon induced showers start a bit later than electron induced, showers (initial pair production has to occur)
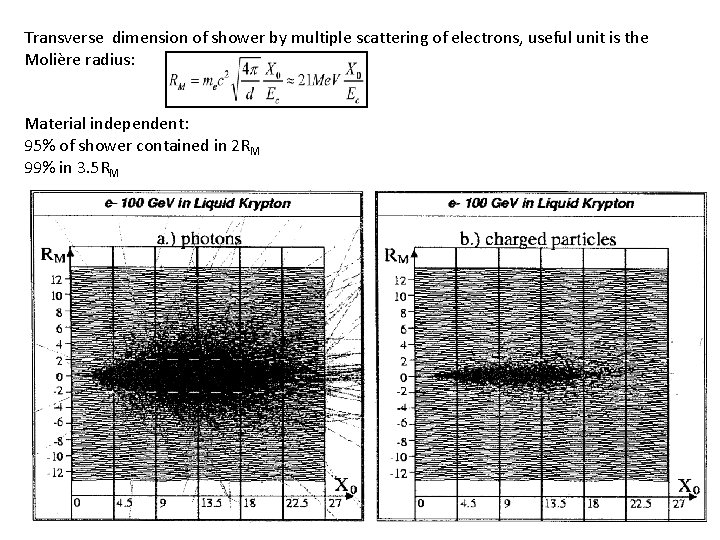
Transverse dimension of shower by multiple scattering of electrons, useful unit is the Molière radius: Material independent: 95% of shower contained in 2 RM 99% in 3. 5 RM
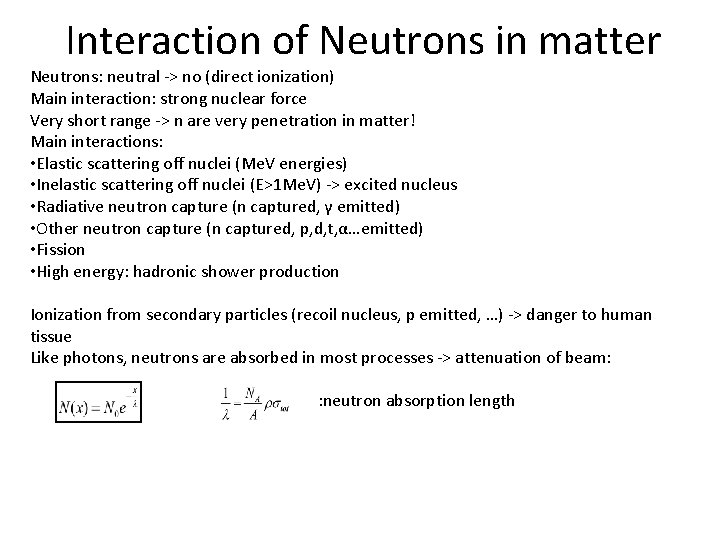
Interaction of Neutrons in matter Neutrons: neutral -> no (direct ionization) Main interaction: strong nuclear force Very short range -> n are very penetration in matter! Main interactions: • Elastic scattering off nuclei (Me. V energies) • Inelastic scattering off nuclei (E>1 Me. V) -> excited nucleus • Radiative neutron capture (n captured, γ emitted) • Other neutron capture (n captured, p, d, t, α…emitted) • Fission • High energy: hadronic shower production Ionization from secondary particles (recoil nucleus, p emitted, …) -> danger to human tissue Like photons, neutrons are absorbed in most processes -> attenuation of beam: : neutron absorption length
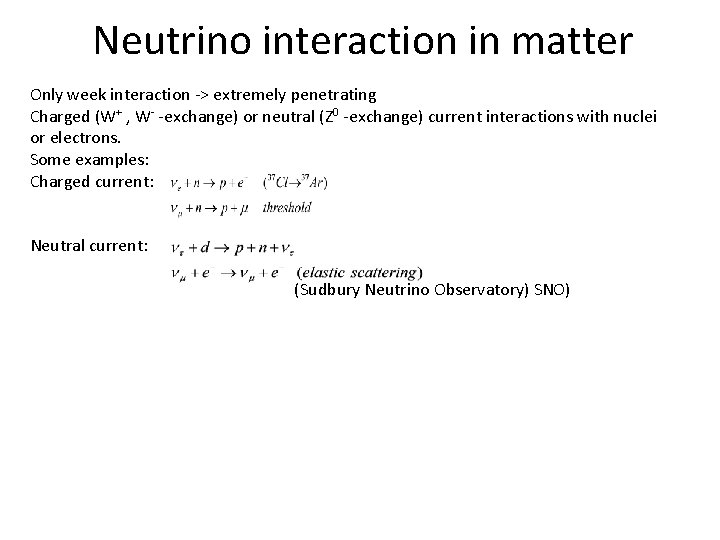
Neutrino interaction in matter Only week interaction -> extremely penetrating Charged (W+ , W- -exchange) or neutral (Z 0 -exchange) current interactions with nuclei or electrons. Some examples: Charged current: Neutral current: (Sudbury Neutrino Observatory) SNO)
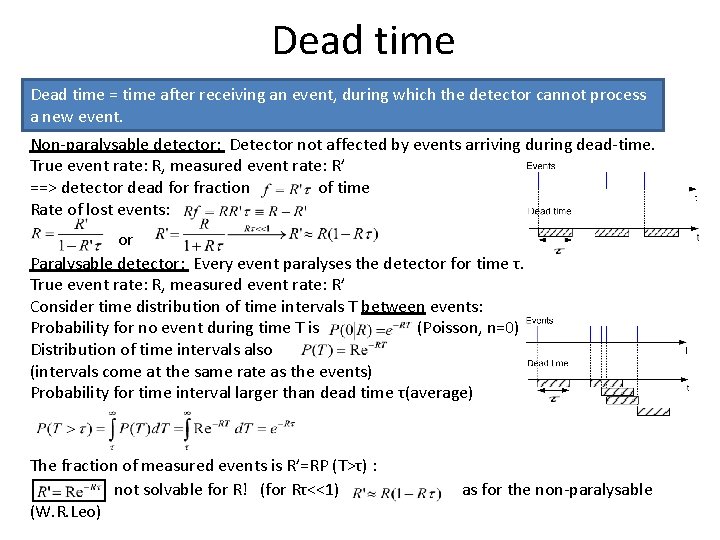
Dead time = time after receiving an event, during which the detector cannot process a new event. Non-paralysable detector: Detector not affected by events arriving during dead-time. True event rate: R, measured event rate: R’ ==> detector dead for fraction of time Rate of lost events: or Paralysable detector: Every event paralyses the detector for time τ. True event rate: R, measured event rate: R’ Consider time distribution of time intervals T between events: Probability for no event during time T is (Poisson, n=0) Distribution of time intervals also (intervals come at the same rate as the events) Probability for time interval larger than dead time τ(average) The fraction of measured events is R’=RP (T>τ) : not solvable for R! (for Rτ<<1) (W. R. Leo) as for the non-paralysable
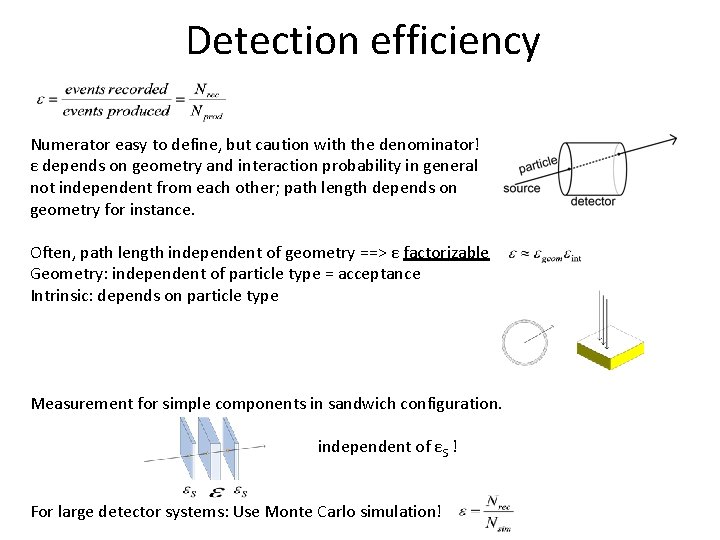
Detection efficiency Numerator easy to define, but caution with the denominator! ε depends on geometry and interaction probability in general not independent from each other; path length depends on geometry for instance. Often, path length independent of geometry ==> ε factorizable Geometry: independent of particle type = acceptance Intrinsic: depends on particle type Measurement for simple components in sandwich configuration. independent of εS ! For large detector systems: Use Monte Carlo simulation!
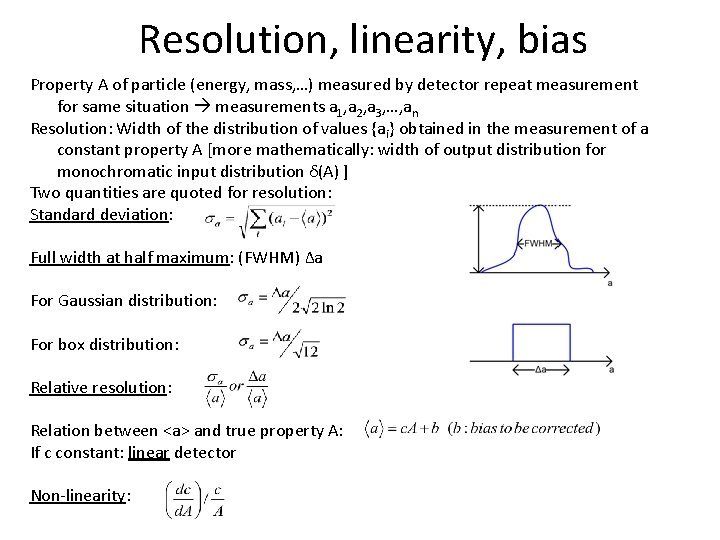
Resolution, linearity, bias Property A of particle (energy, mass, …) measured by detector repeat measurement for same situation measurements a 1, a 2, a 3, …, an Resolution: Width of the distribution of values {ai} obtained in the measurement of a constant property A [more mathematically: width of output distribution for monochromatic input distribution δ(A) ] Two quantities are quoted for resolution: Standard deviation: Full width at half maximum: (FWHM) Δa For Gaussian distribution: For box distribution: Relative resolution: Relation between <a> and true property A: If c constant: linear detector Non-linearity:
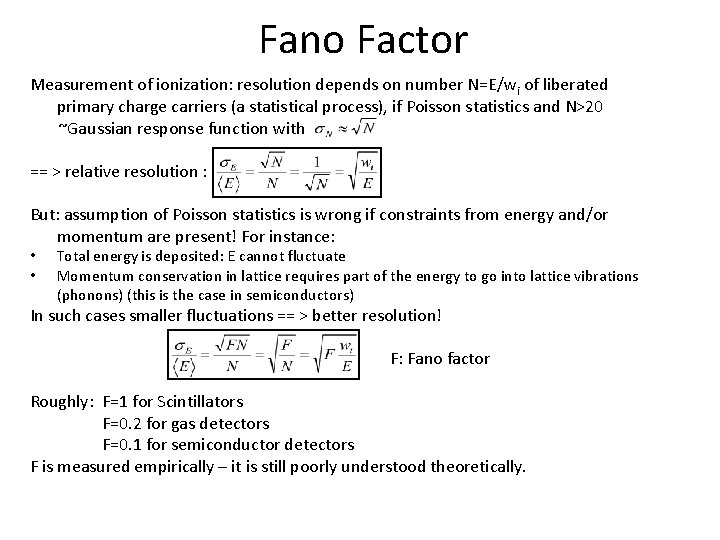
Fano Factor Measurement of ionization: resolution depends on number N=E/wi of liberated primary charge carriers (a statistical process), if Poisson statistics and N>20 ~Gaussian response function with == > relative resolution : But: assumption of Poisson statistics is wrong if constraints from energy and/or momentum are present! For instance: • • Total energy is deposited: E cannot fluctuate Momentum conservation in lattice requires part of the energy to go into lattice vibrations (phonons) (this is the case in semiconductors) In such cases smaller fluctuations == > better resolution! F: Fano factor Roughly: F=1 for Scintillators F=0. 2 for gas detectors F=0. 1 for semiconductor detectors F is measured empirically – it is still poorly understood theoretically.
Facts of light
Quantum imaging with undetected photons
"perkin elmer"
Momentum of photon
What is a photon made of
01:640:244 lecture notes - lecture 15: plat, idah, farad
Time varying fields and maxwell's equations
Low frequency attenuation
Pain attenuation definition
Ultrasound beam attenuation
Attenuation x ray