Equation of motion of charged particles the Lorentz
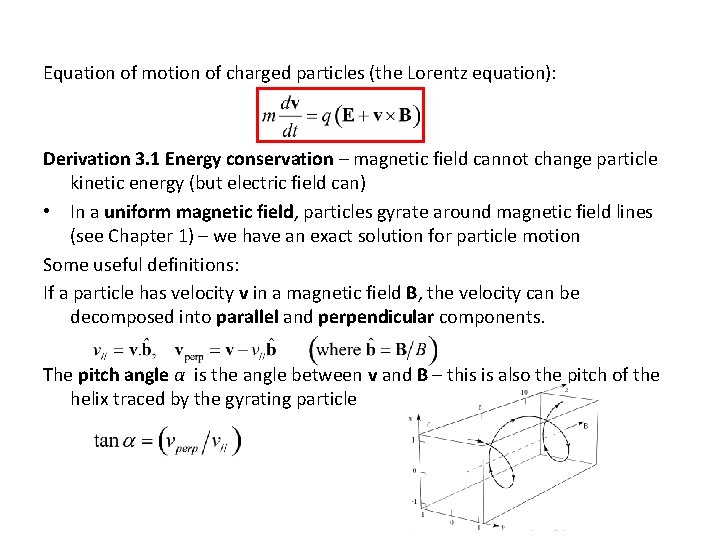
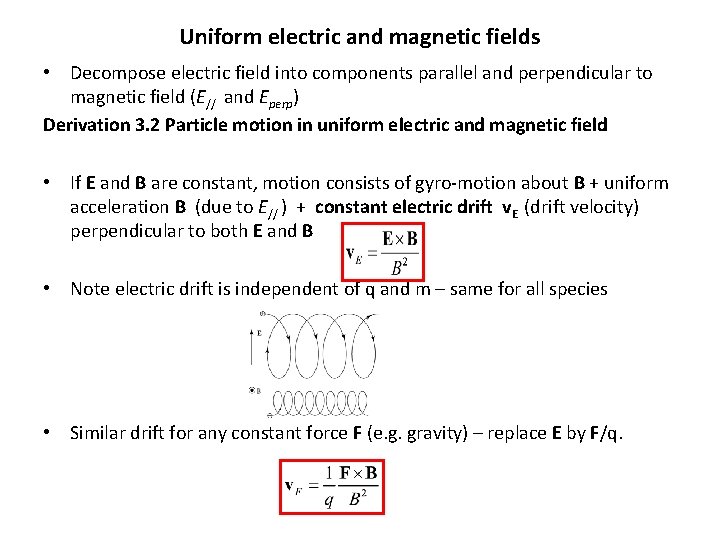
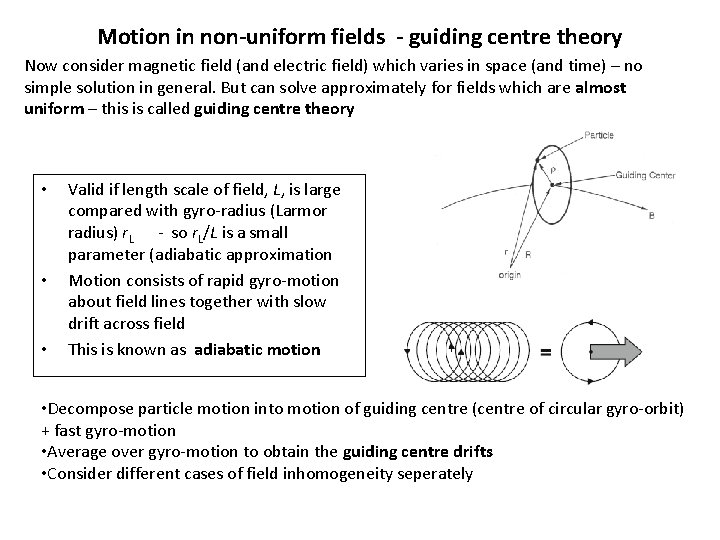
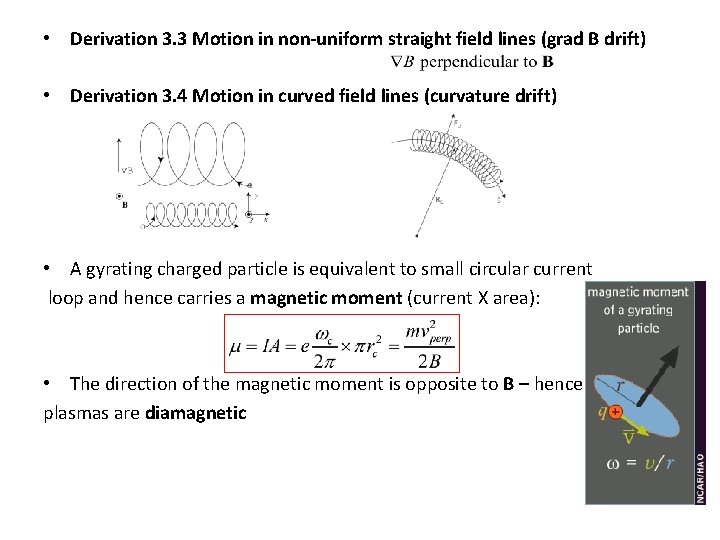
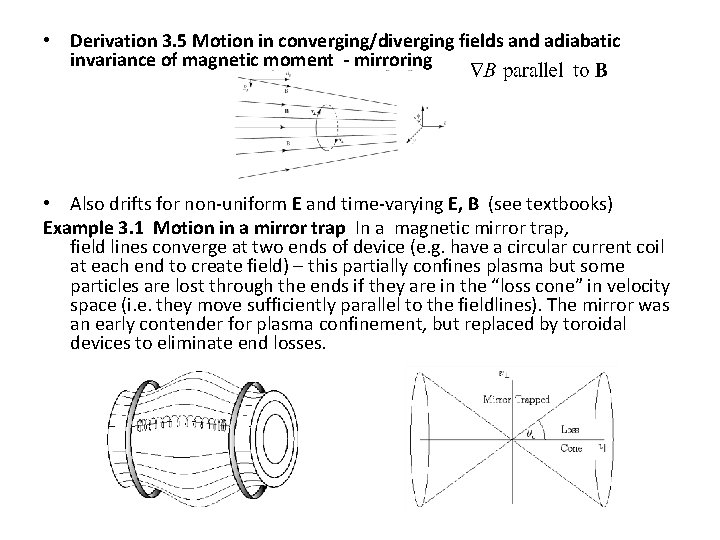
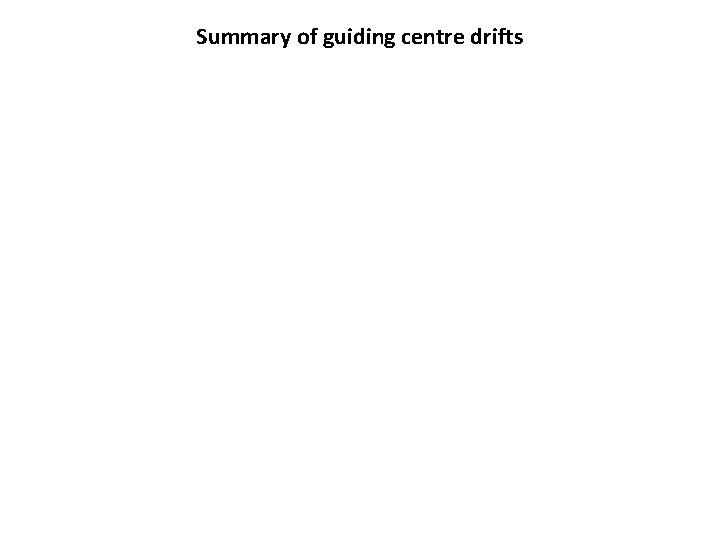
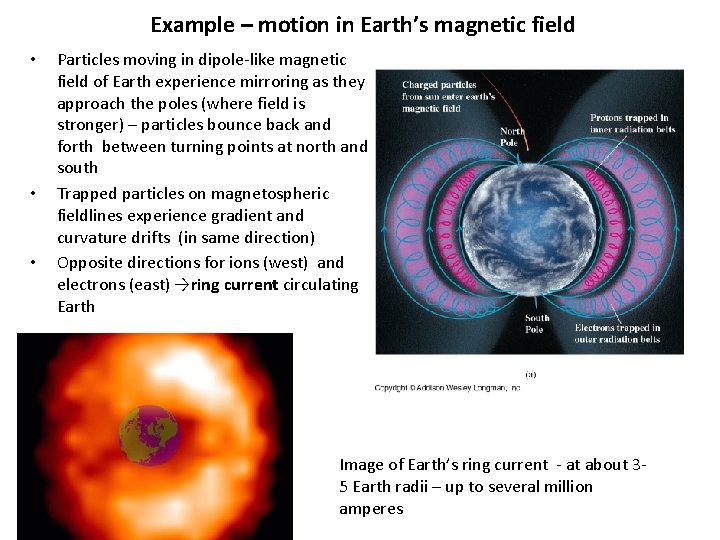
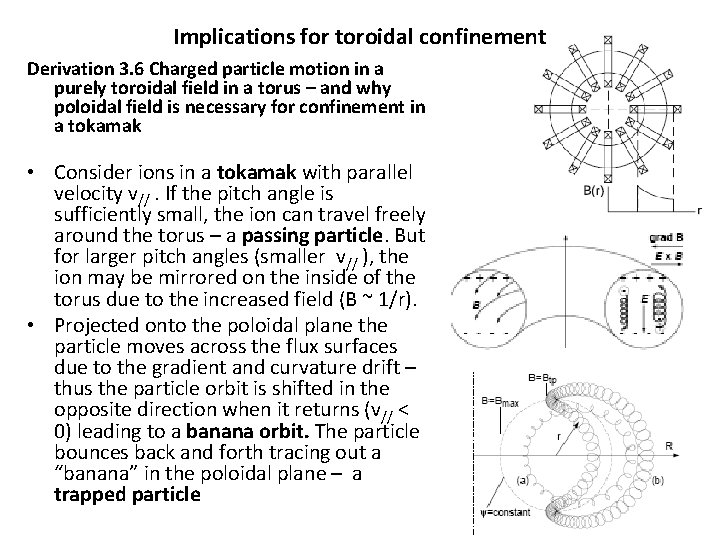
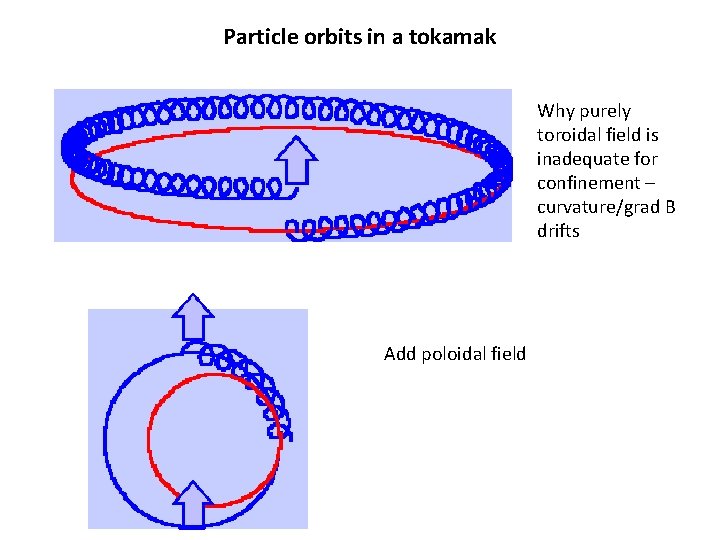
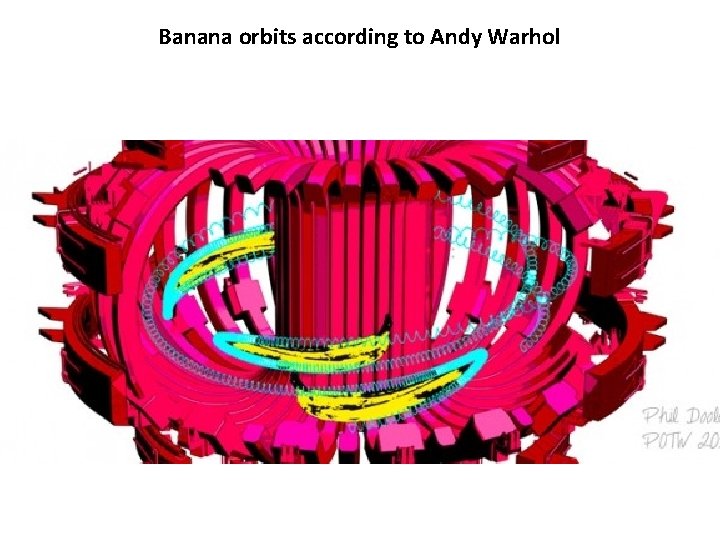
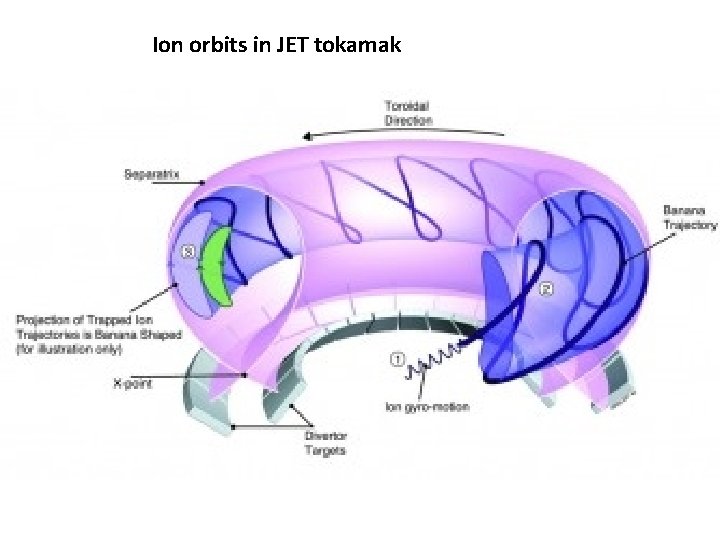
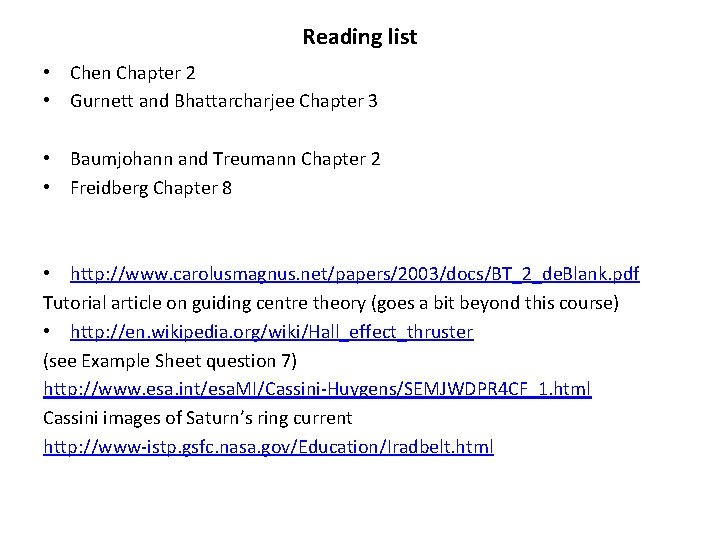
- Slides: 12
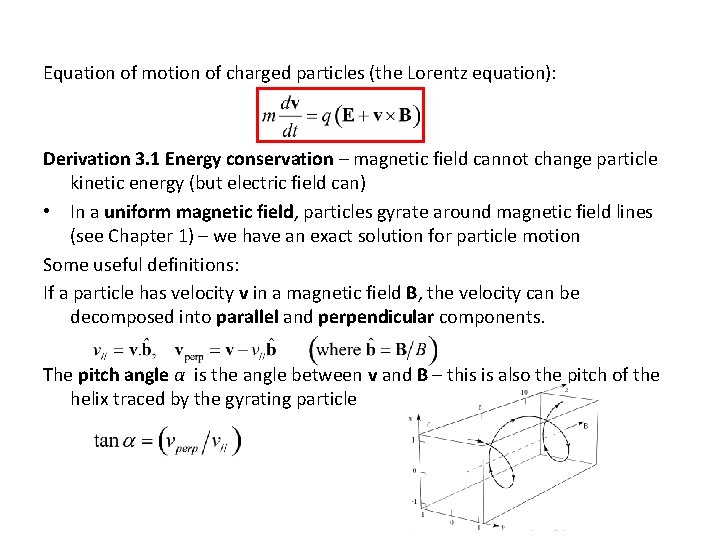
Equation of motion of charged particles (the Lorentz equation): Derivation 3. 1 Energy conservation – magnetic field cannot change particle kinetic energy (but electric field can) • In a uniform magnetic field, particles gyrate around magnetic field lines (see Chapter 1) – we have an exact solution for particle motion Some useful definitions: If a particle has velocity v in a magnetic field B, the velocity can be decomposed into parallel and perpendicular components. The pitch angle α is the angle between v and B – this is also the pitch of the helix traced by the gyrating particle
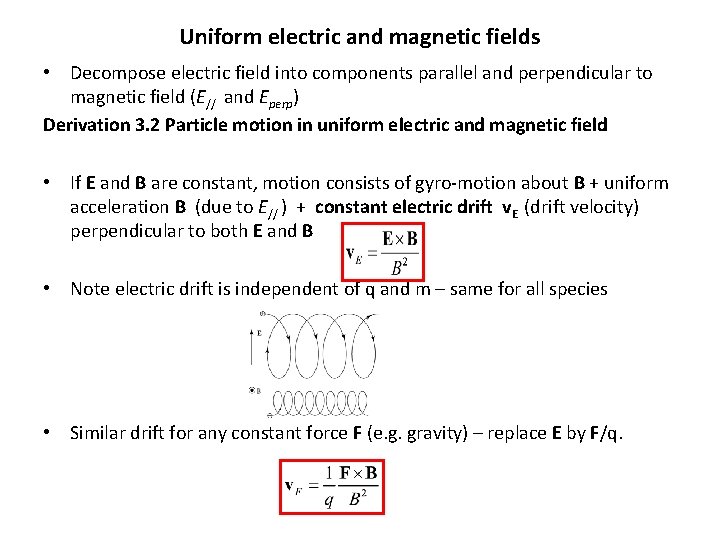
Uniform electric and magnetic fields • Decompose electric field into components parallel and perpendicular to magnetic field (E// and Eperp) Derivation 3. 2 Particle motion in uniform electric and magnetic field • If E and B are constant, motion consists of gyro-motion about B + uniform acceleration B (due to E// ) + constant electric drift v. E (drift velocity) perpendicular to both E and B • Note electric drift is independent of q and m – same for all species • Similar drift for any constant force F (e. g. gravity) – replace E by F/q.
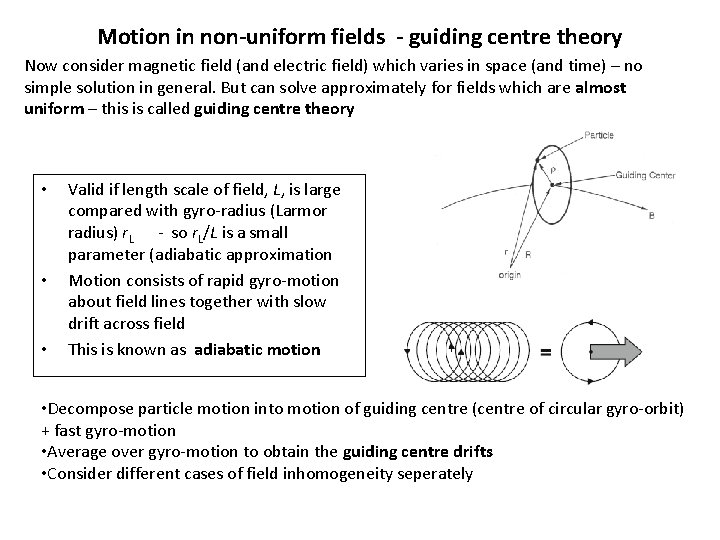
Motion in non-uniform fields - guiding centre theory Now consider magnetic field (and electric field) which varies in space (and time) – no simple solution in general. But can solve approximately for fields which are almost uniform – this is called guiding centre theory • • • Valid if length scale of field, L, is large compared with gyro-radius (Larmor radius) r. L - so r. L/L is a small parameter (adiabatic approximation Motion consists of rapid gyro-motion about field lines together with slow drift across field This is known as adiabatic motion • Decompose particle motion into motion of guiding centre (centre of circular gyro-orbit) + fast gyro-motion • Average over gyro-motion to obtain the guiding centre drifts • Consider different cases of field inhomogeneity seperately
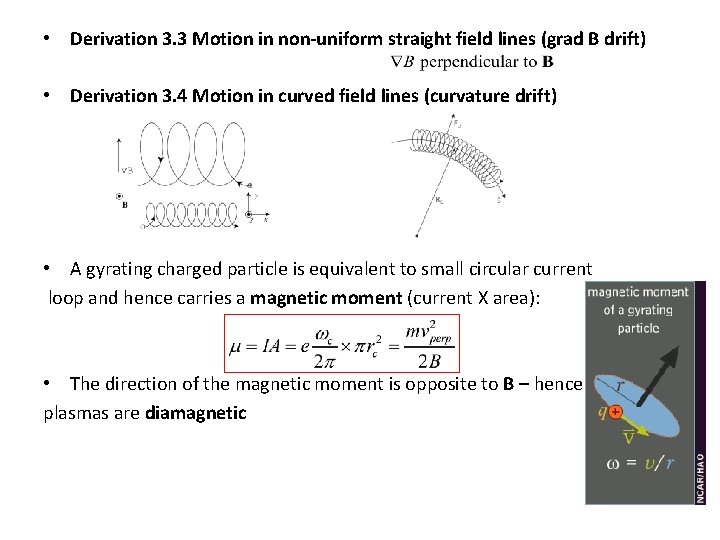
• Derivation 3. 3 Motion in non-uniform straight field lines (grad B drift) • Derivation 3. 4 Motion in curved field lines (curvature drift) • A gyrating charged particle is equivalent to small circular current loop and hence carries a magnetic moment (current X area): • The direction of the magnetic moment is opposite to B – hence plasmas are diamagnetic
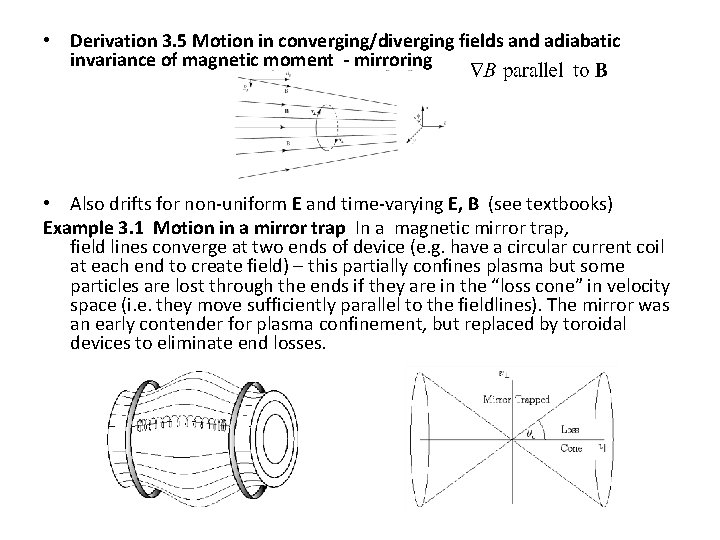
• Derivation 3. 5 Motion in converging/diverging fields and adiabatic invariance of magnetic moment - mirroring • Also drifts for non-uniform E and time-varying E, B (see textbooks) Example 3. 1 Motion in a mirror trap In a magnetic mirror trap, field lines converge at two ends of device (e. g. have a circular current coil at each end to create field) – this partially confines plasma but some particles are lost through the ends if they are in the “loss cone” in velocity space (i. e. they move sufficiently parallel to the fieldlines). The mirror was an early contender for plasma confinement, but replaced by toroidal devices to eliminate end losses.
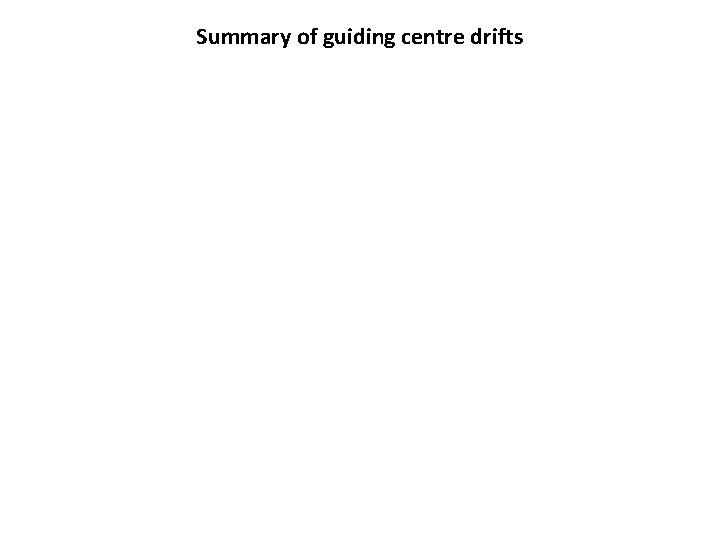
Summary of guiding centre drifts
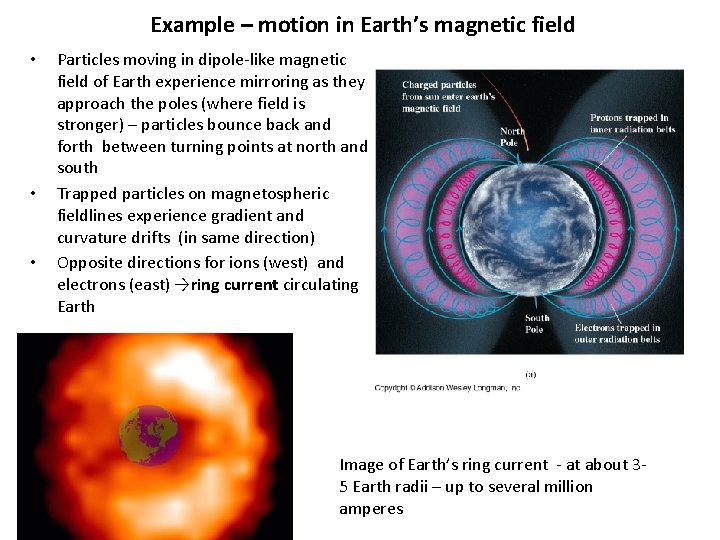
Example – motion in Earth’s magnetic field • • • Particles moving in dipole-like magnetic field of Earth experience mirroring as they approach the poles (where field is stronger) – particles bounce back and forth between turning points at north and south Trapped particles on magnetospheric fieldlines experience gradient and curvature drifts (in same direction) Opposite directions for ions (west) and electrons (east) →ring current circulating Earth Image of Earth’s ring current - at about 35 Earth radii – up to several million amperes
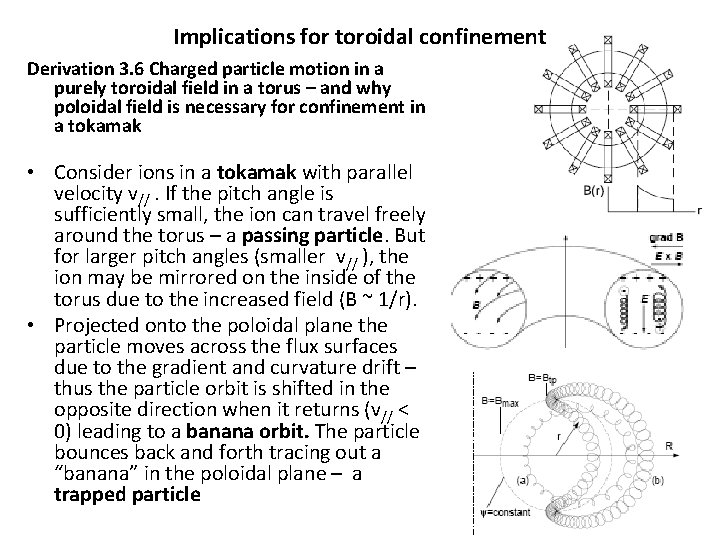
Implications for toroidal confinement Derivation 3. 6 Charged particle motion in a purely toroidal field in a torus – and why poloidal field is necessary for confinement in a tokamak • Consider ions in a tokamak with parallel velocity v//. If the pitch angle is sufficiently small, the ion can travel freely around the torus – a passing particle. But for larger pitch angles (smaller v// ), the ion may be mirrored on the inside of the torus due to the increased field (B ~ 1/r). • Projected onto the poloidal plane the particle moves across the flux surfaces due to the gradient and curvature drift – thus the particle orbit is shifted in the opposite direction when it returns (v// < 0) leading to a banana orbit. The particle bounces back and forth tracing out a “banana” in the poloidal plane – a trapped particle
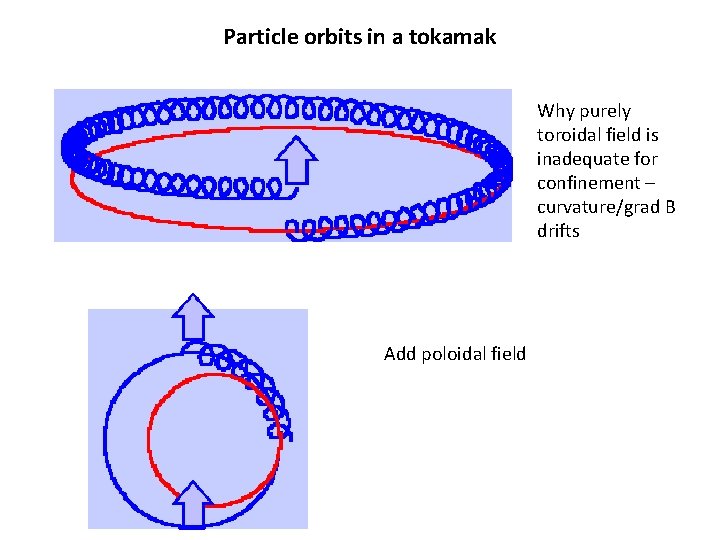
Particle orbits in a tokamak Why purely toroidal field is inadequate for confinement – curvature/grad B drifts Add poloidal field
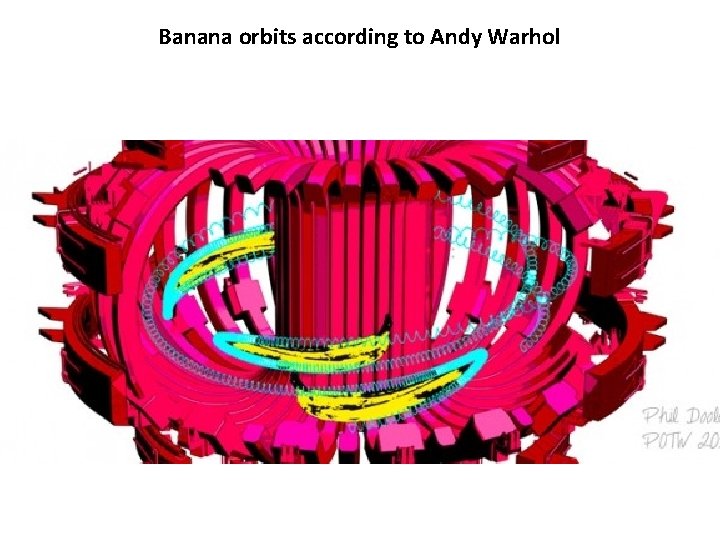
Banana orbits according to Andy Warhol
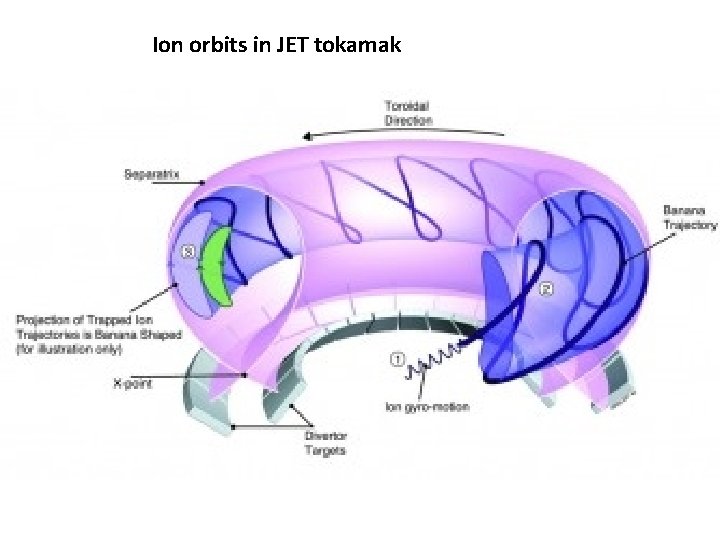
Ion orbits in JET tokamak
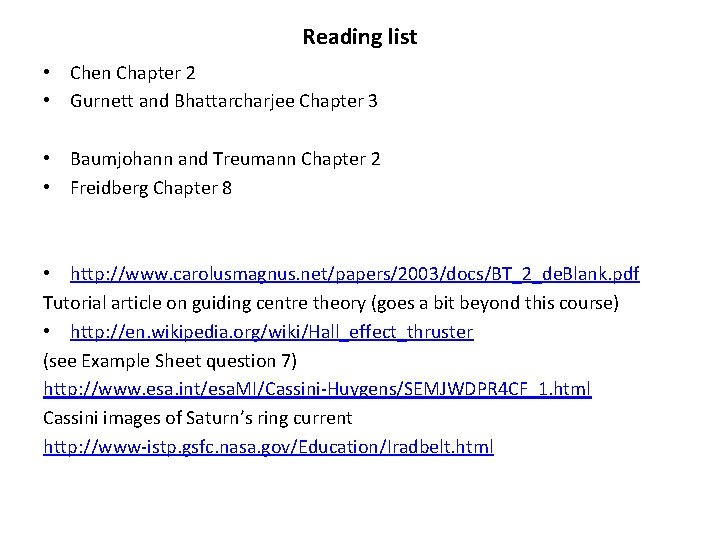
Reading list • Chen Chapter 2 • Gurnett and Bhattarcharjee Chapter 3 • Baumjohann and Treumann Chapter 2 • Freidberg Chapter 8 • http: //www. carolusmagnus. net/papers/2003/docs/BT_2_de. Blank. pdf Tutorial article on guiding centre theory (goes a bit beyond this course) • http: //en. wikipedia. org/wiki/Hall_effect_thruster (see Example Sheet question 7) http: //www. esa. int/esa. MI/Cassini-Huygens/SEMJWDPR 4 CF_1. html Cassini images of Saturn’s ring current http: //www-istp. gsfc. nasa. gov/Education/Iradbelt. html
The search for fractionally charged particles has
Charged particles can be accelerated by
Ions charged particles in solution
For charged particles, what is the quantity q/m called?
Gas like mixture of charged particles
Lorentz transformations
Derive lorentz transformation equation
Lorentz transformation equation derivation
Lorentz transformation equation
What is electromagnetic force
Simple harmonic motion formula
Relative motion of two particles using translating axes
Rectilinear motion of particles