Overview Lifes Operating Instructions In 1953 James Watson
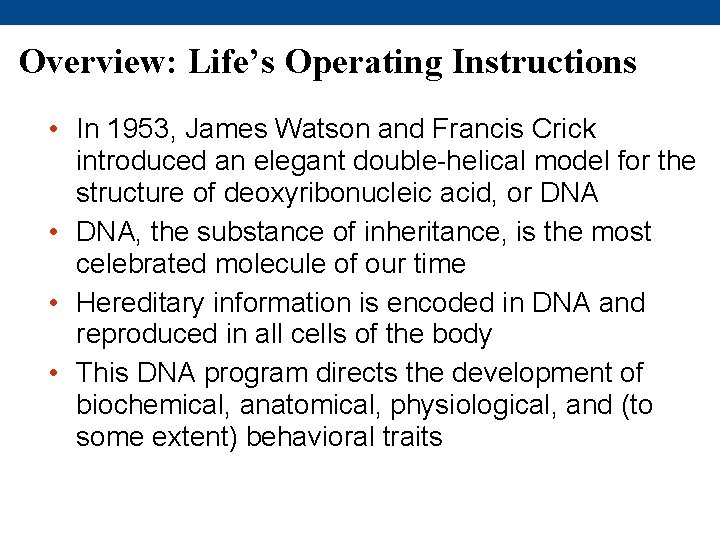
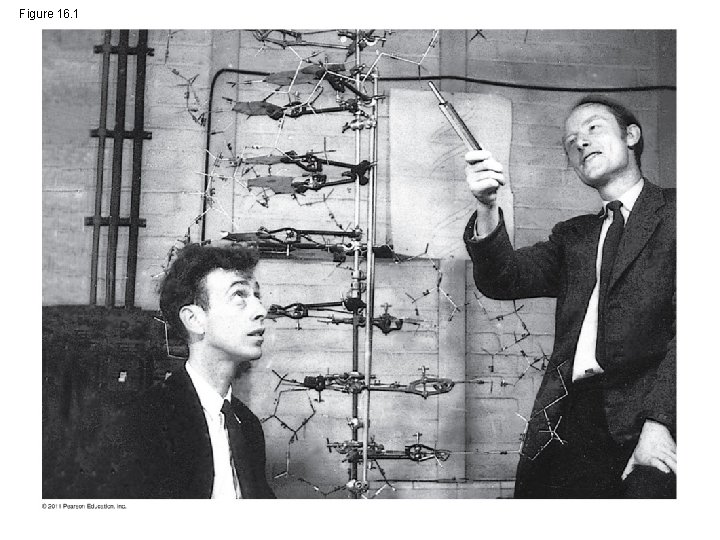
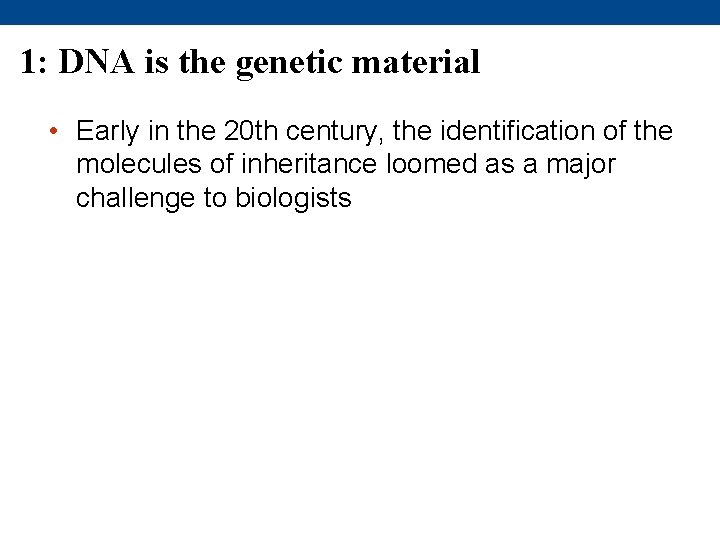
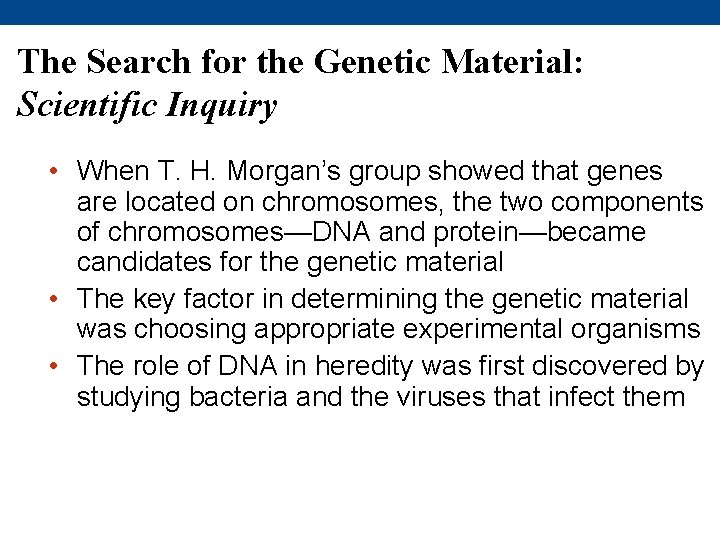
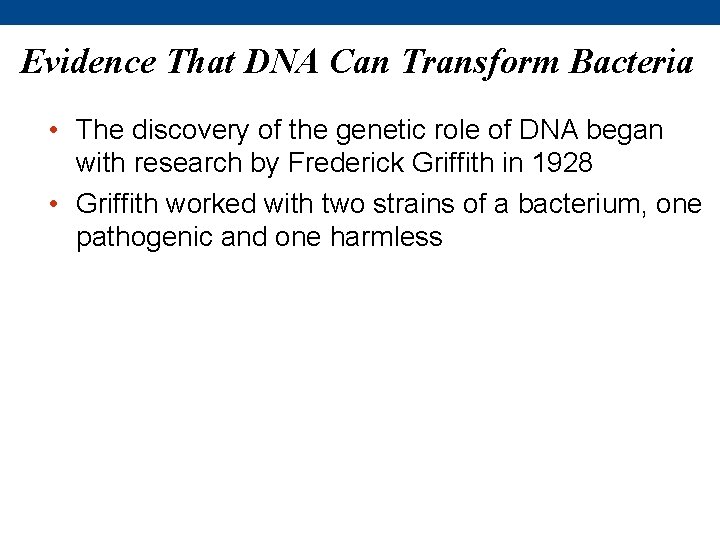
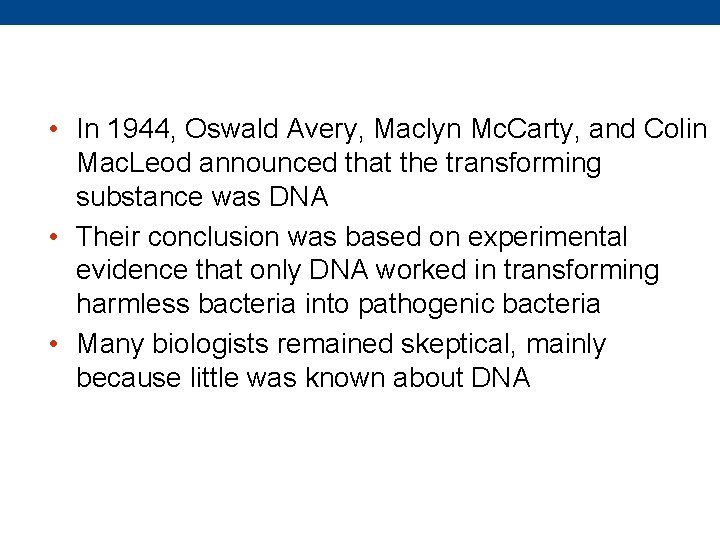
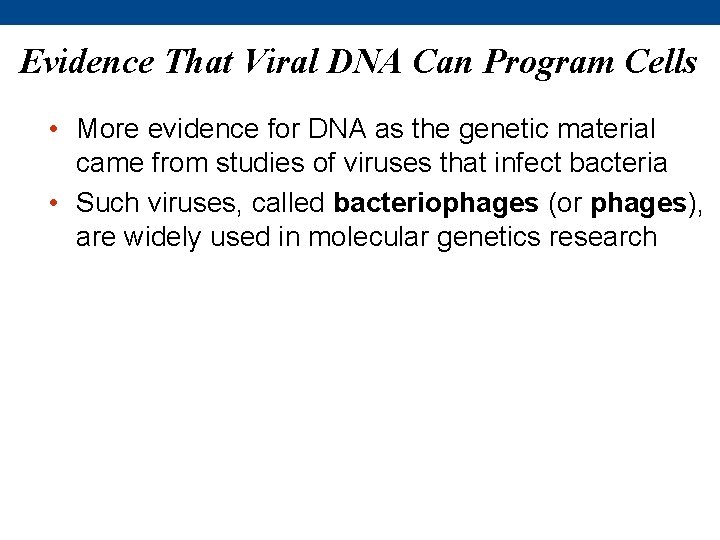
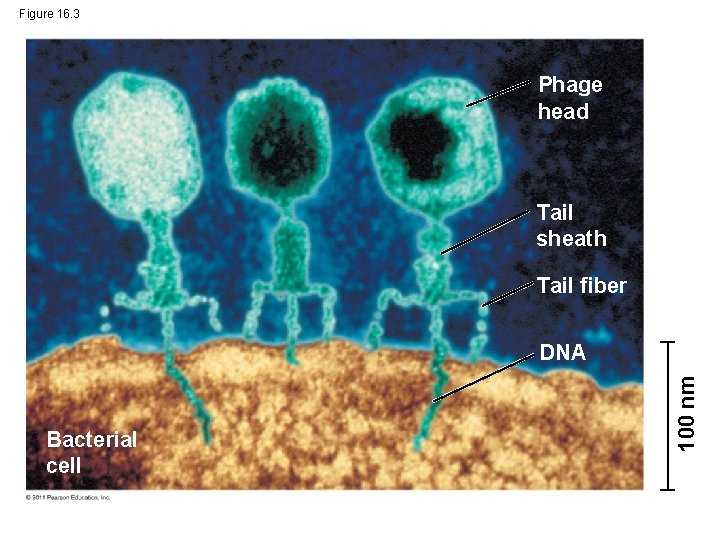
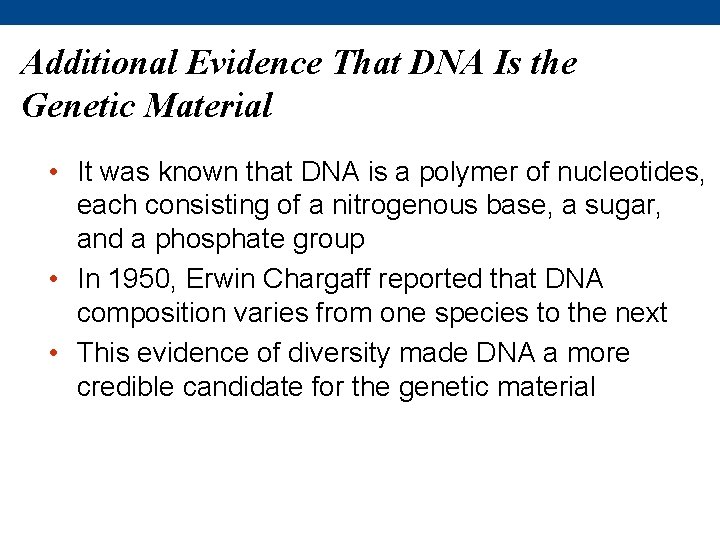
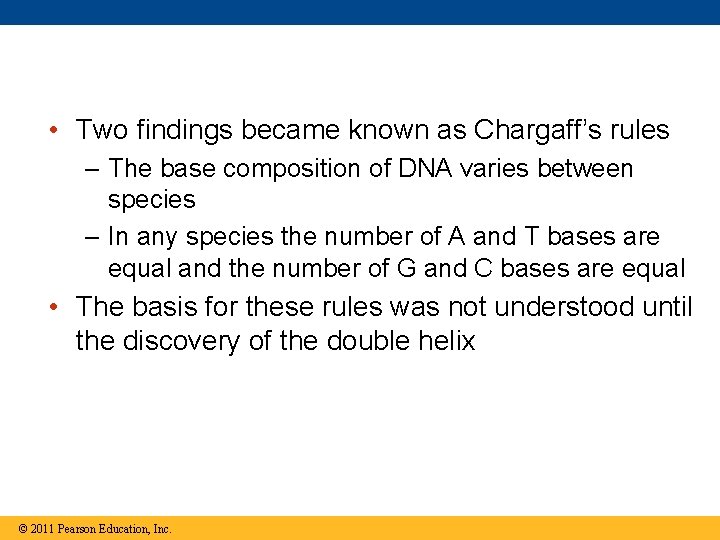
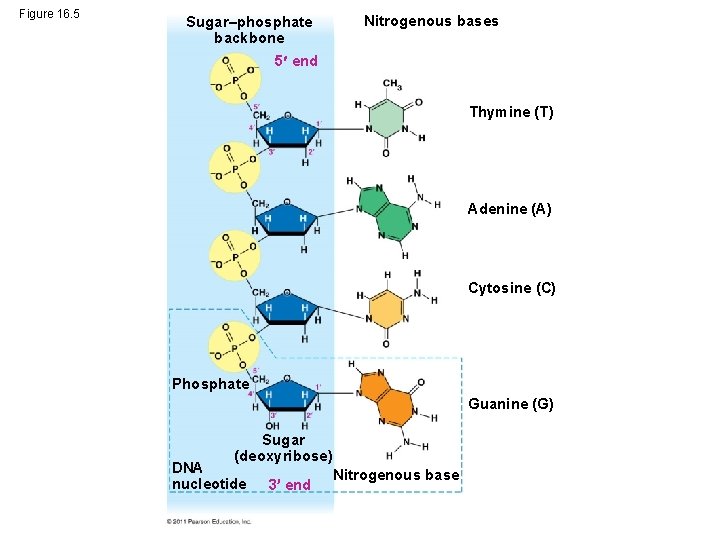
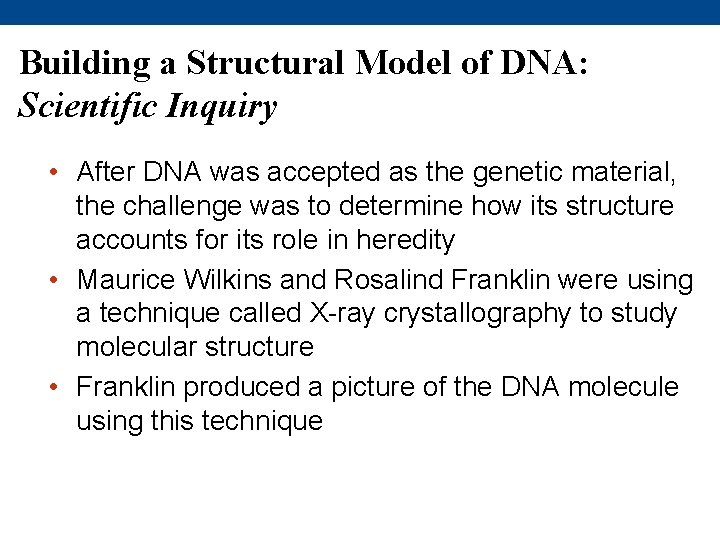
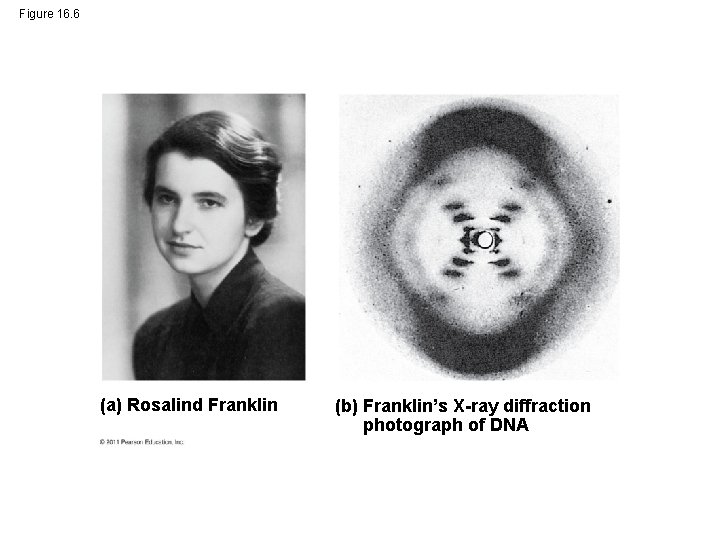
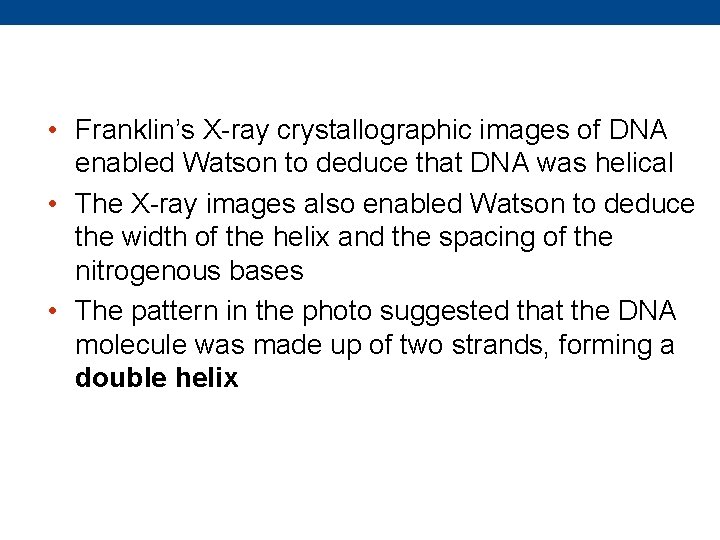
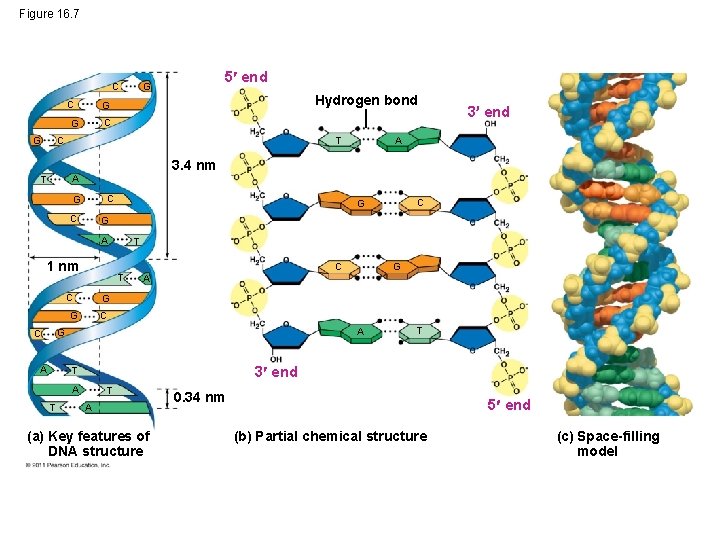
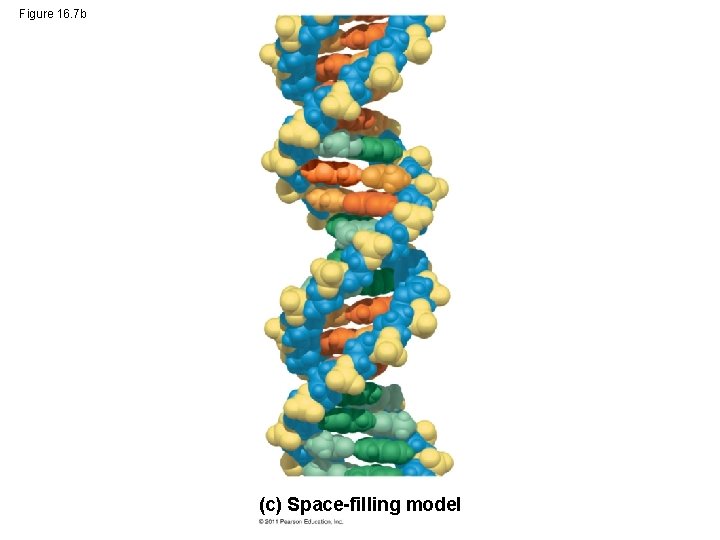
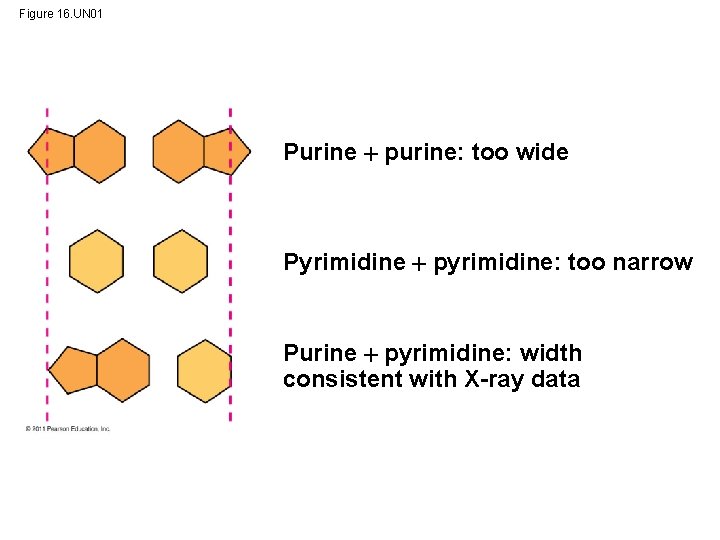
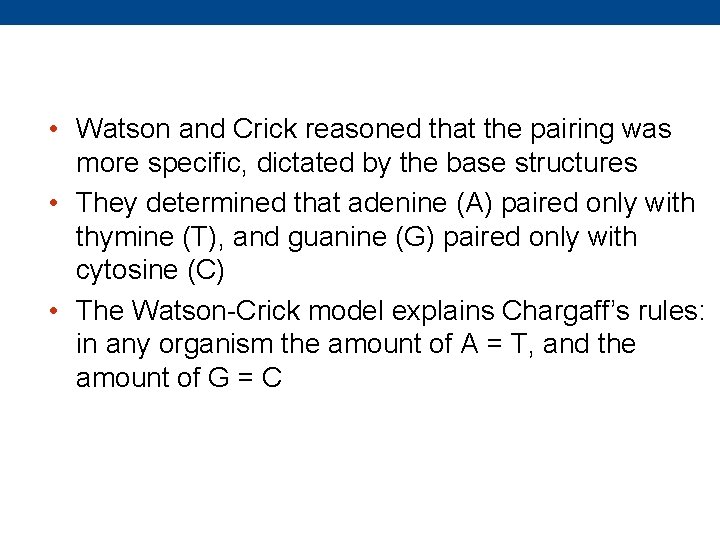
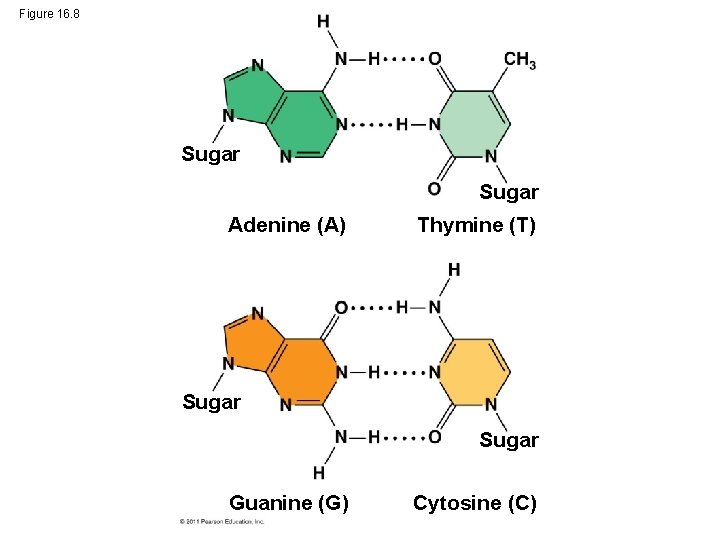
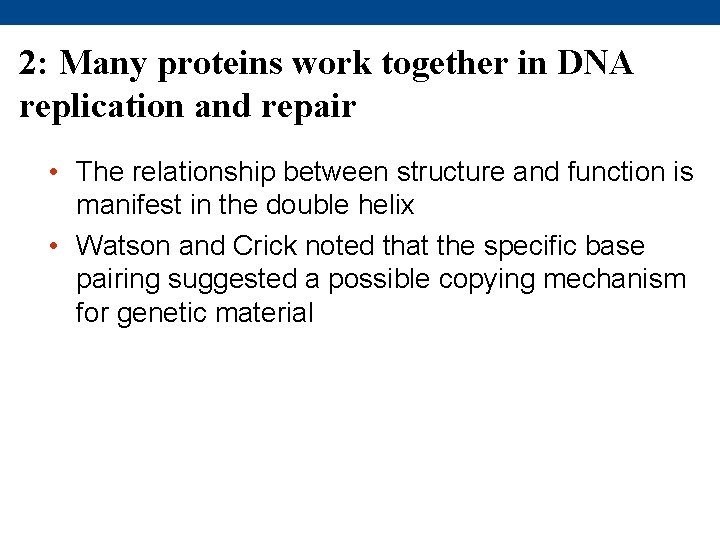
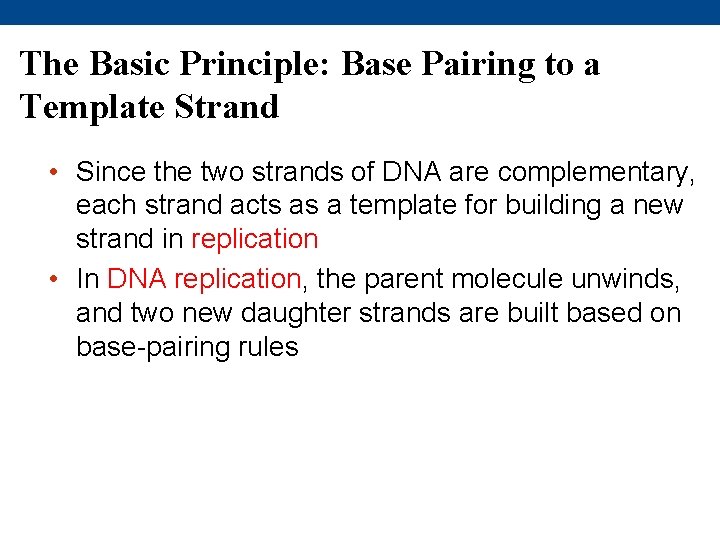
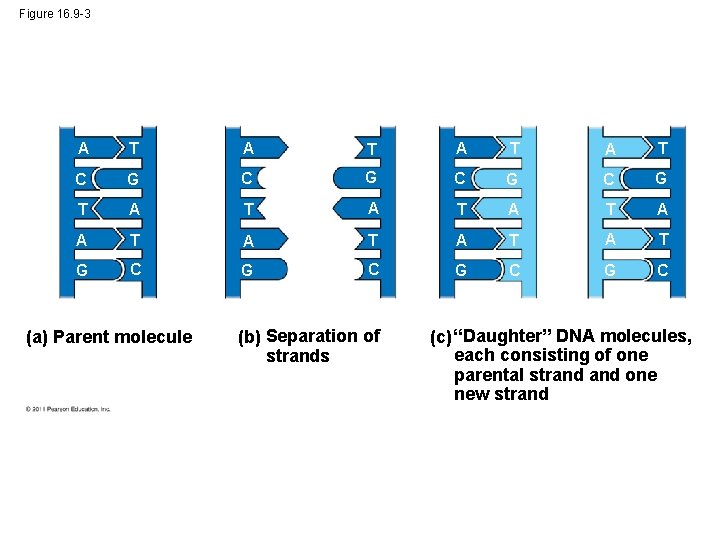
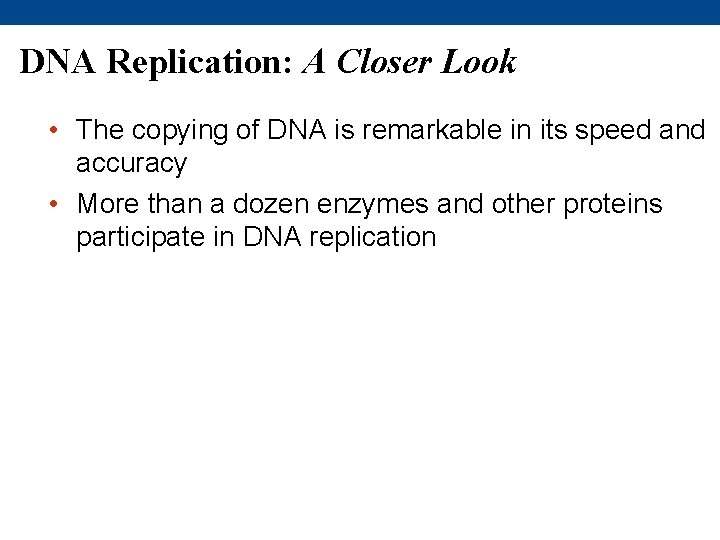
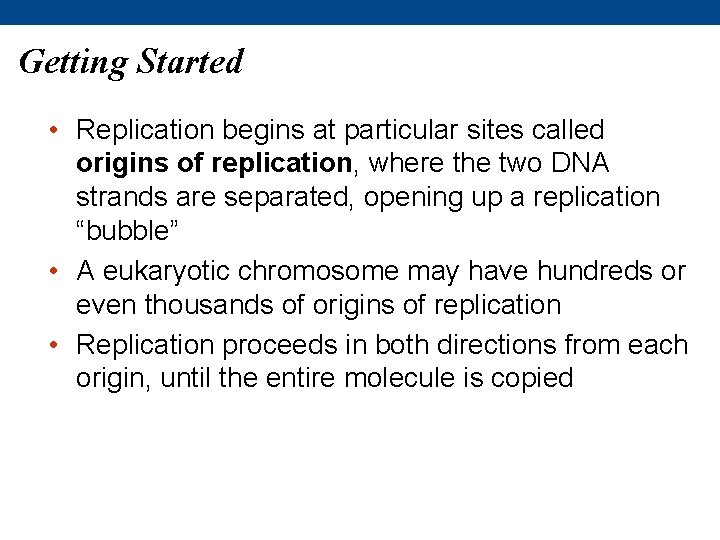
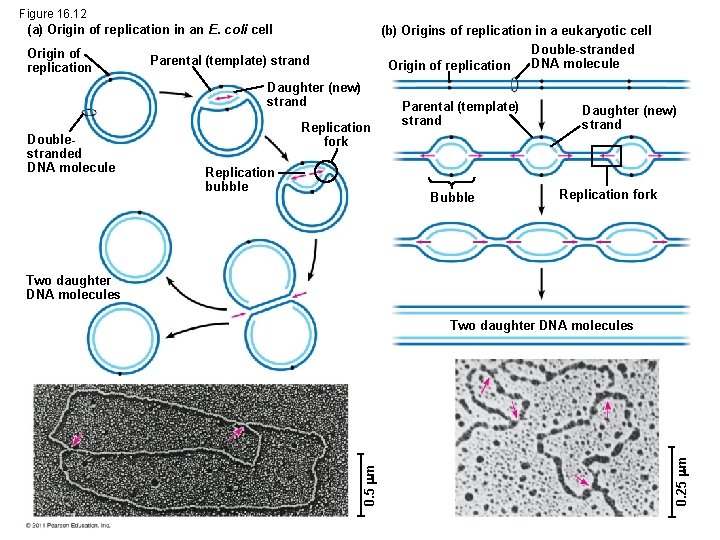
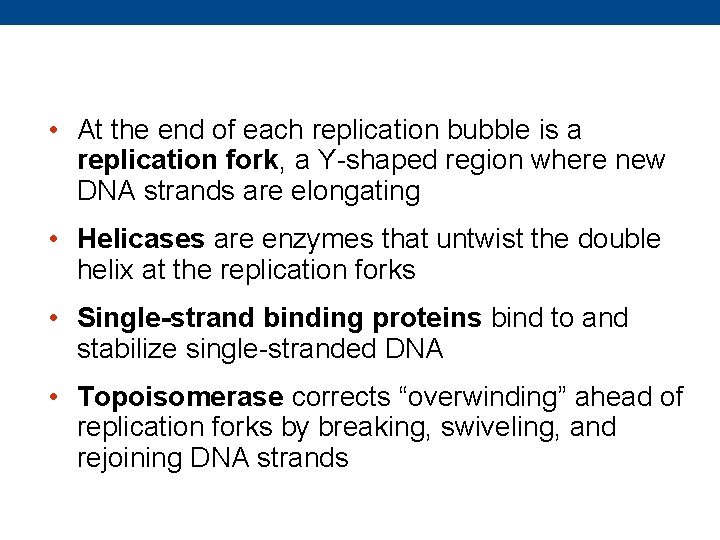
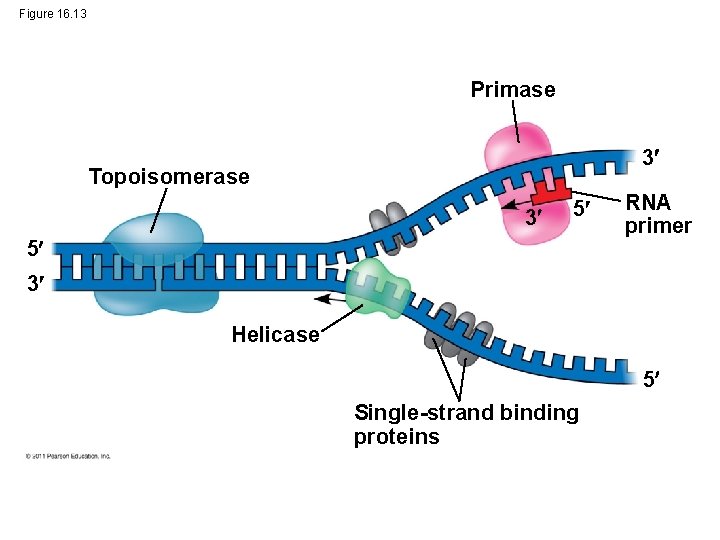
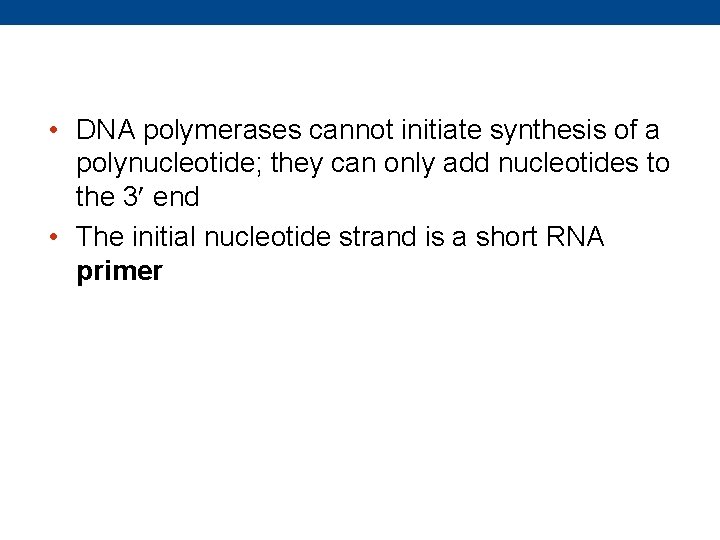
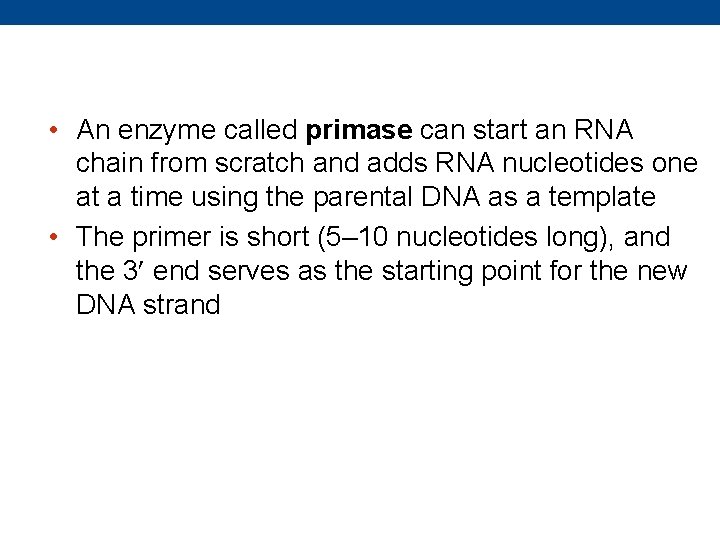
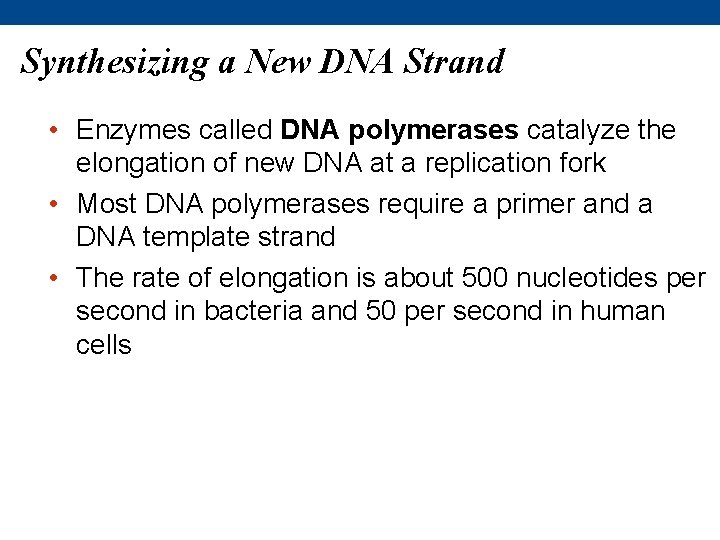
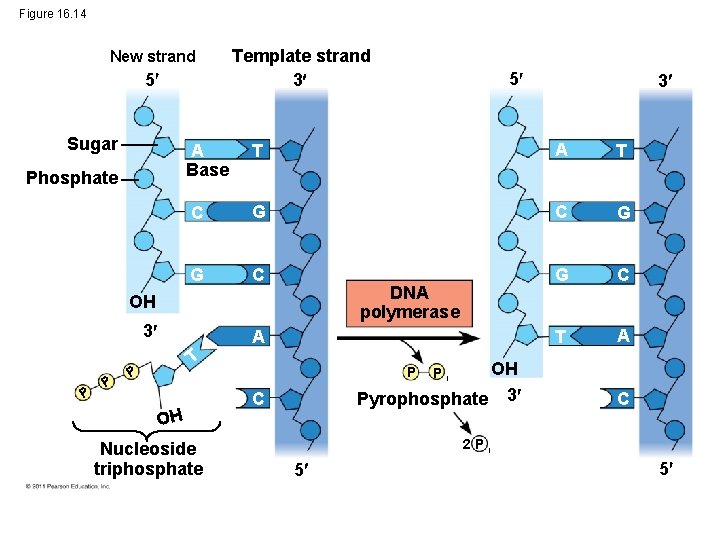
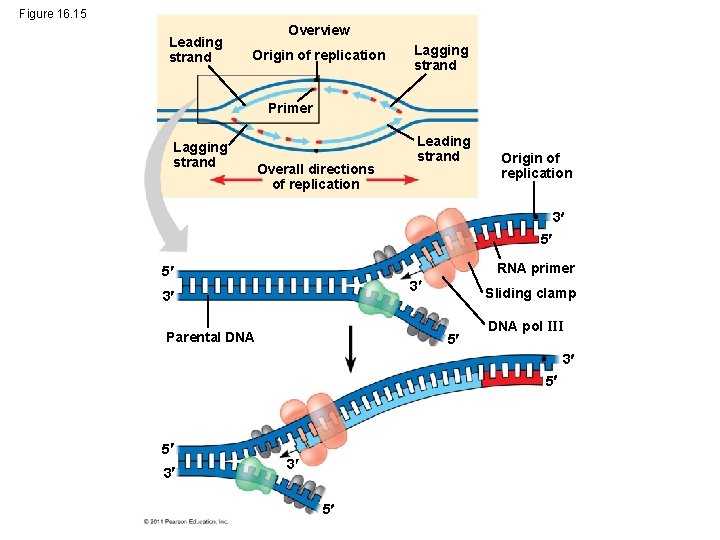
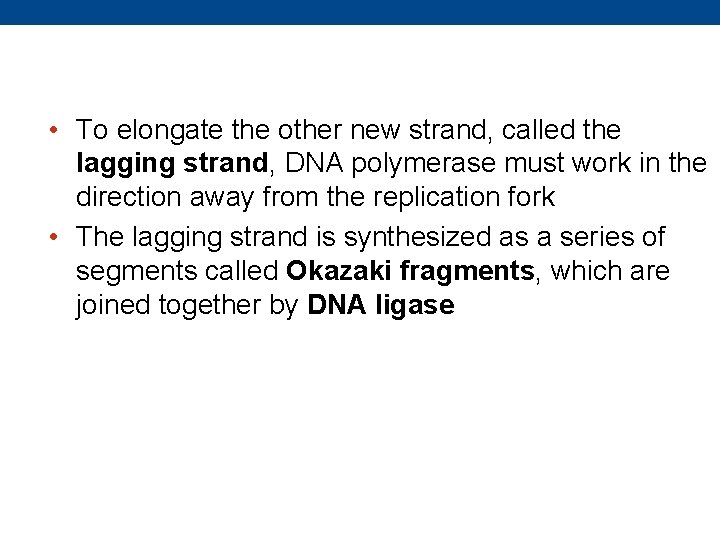
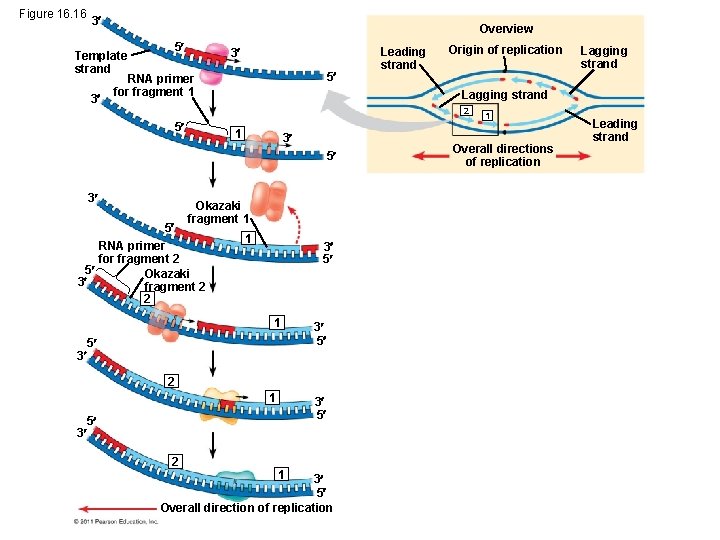
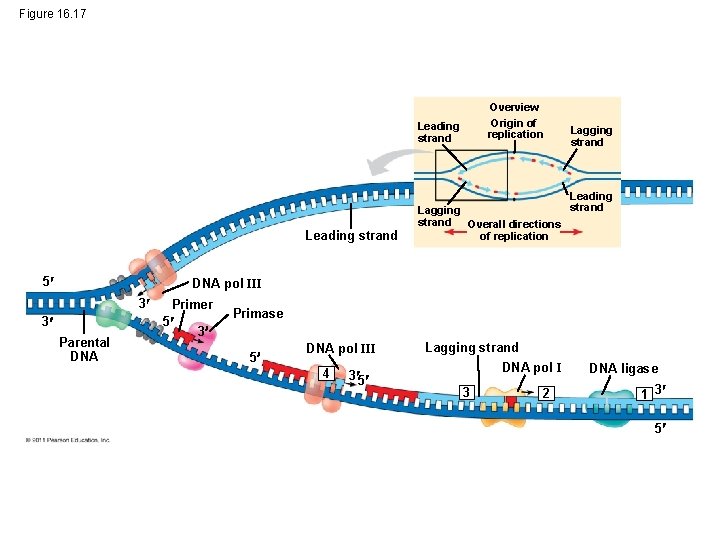
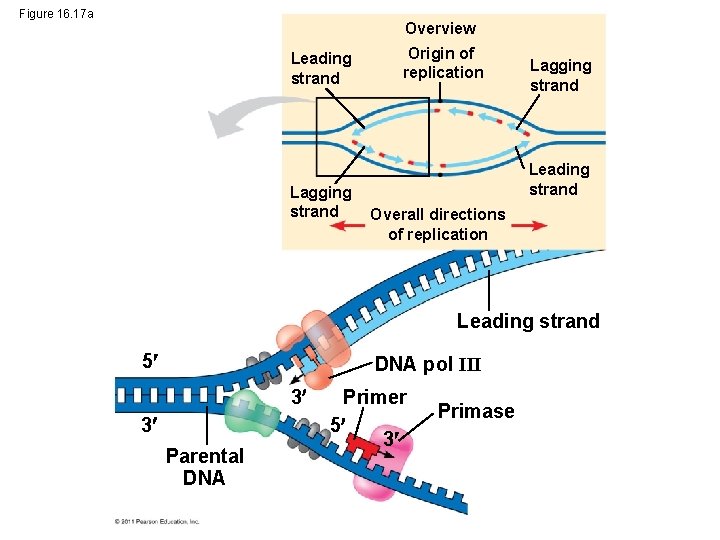
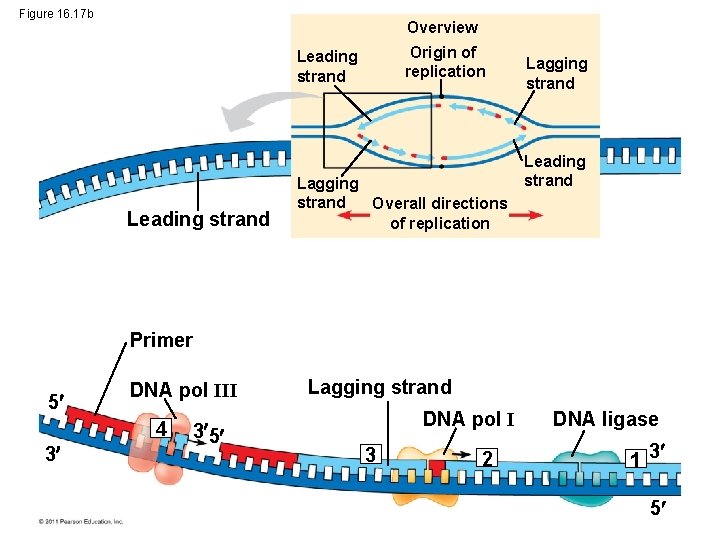
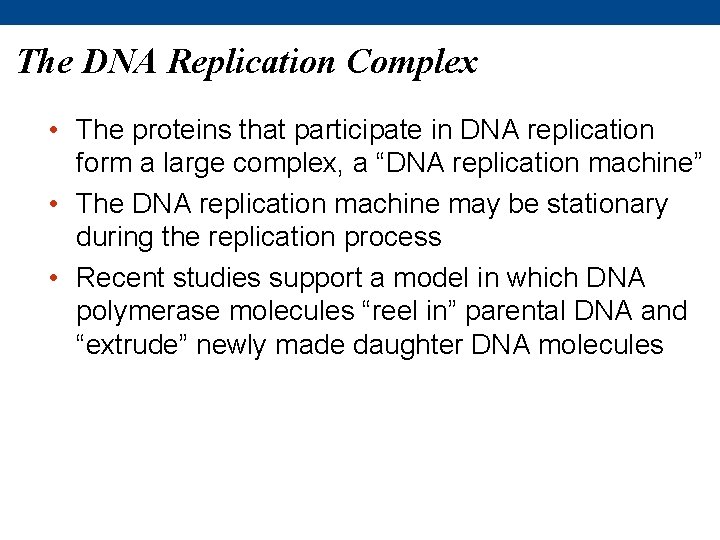
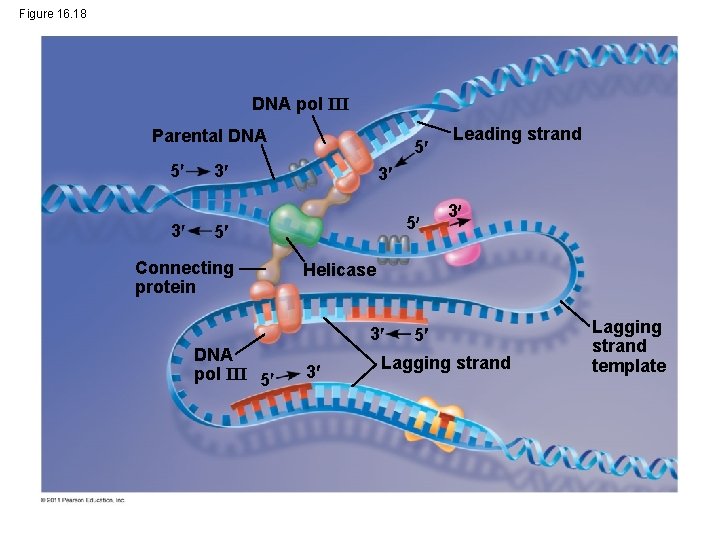
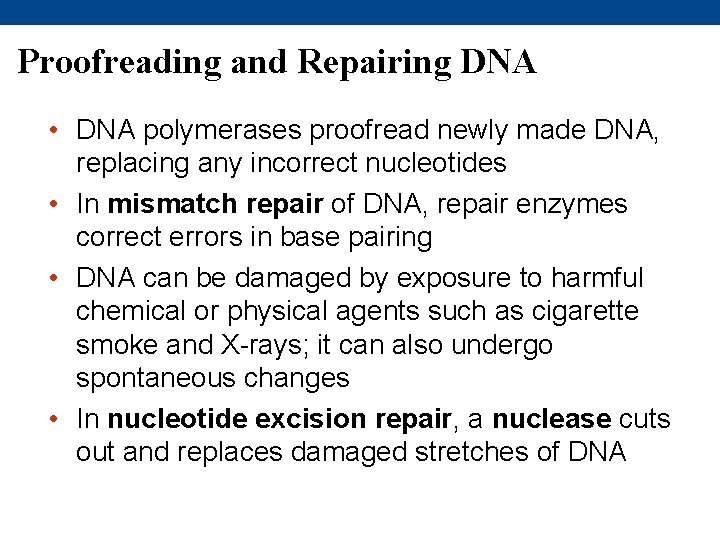
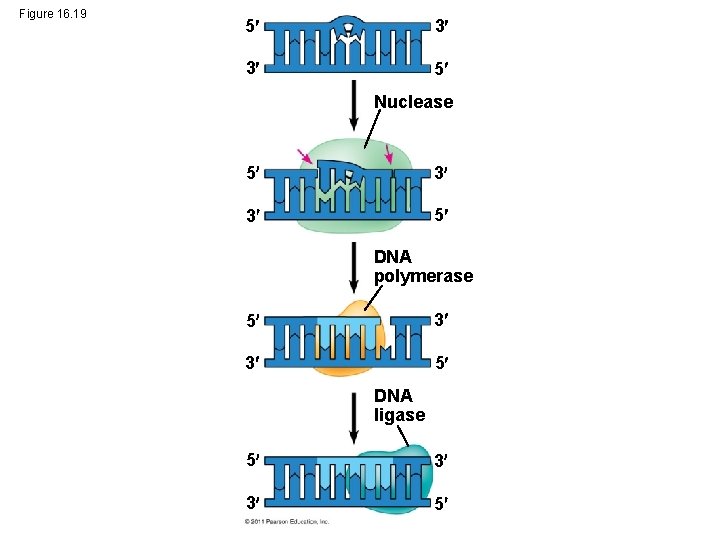
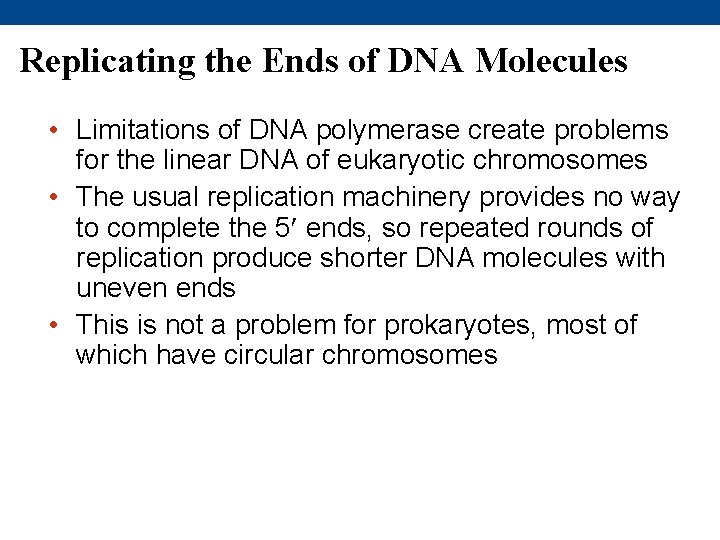
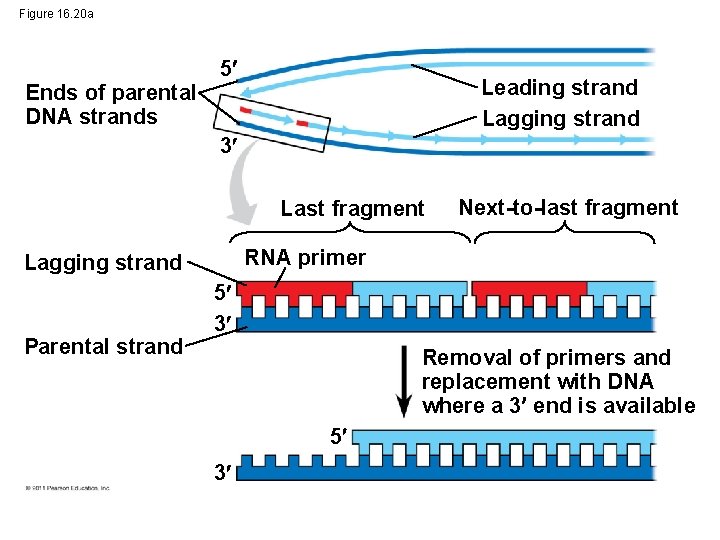
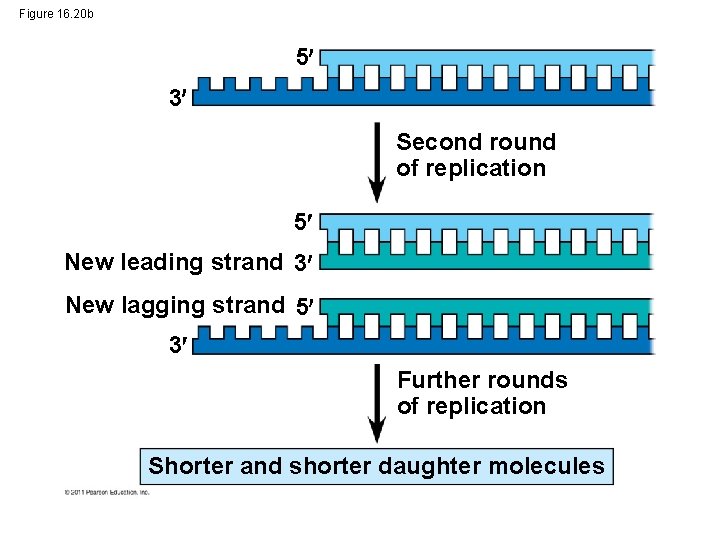
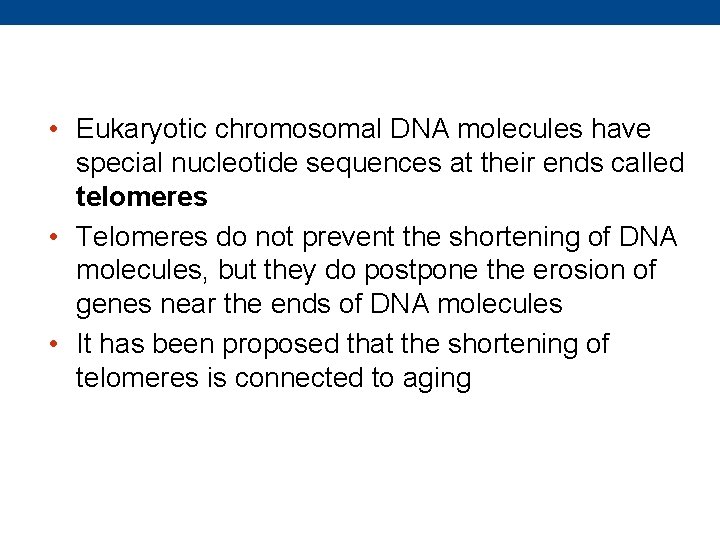
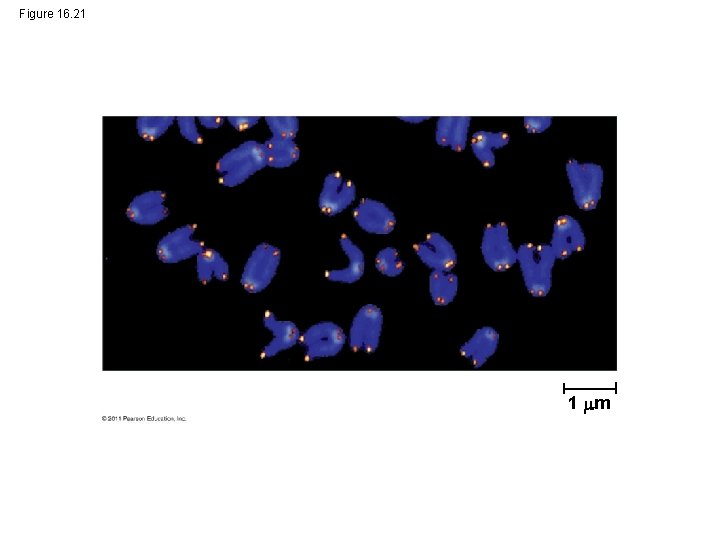
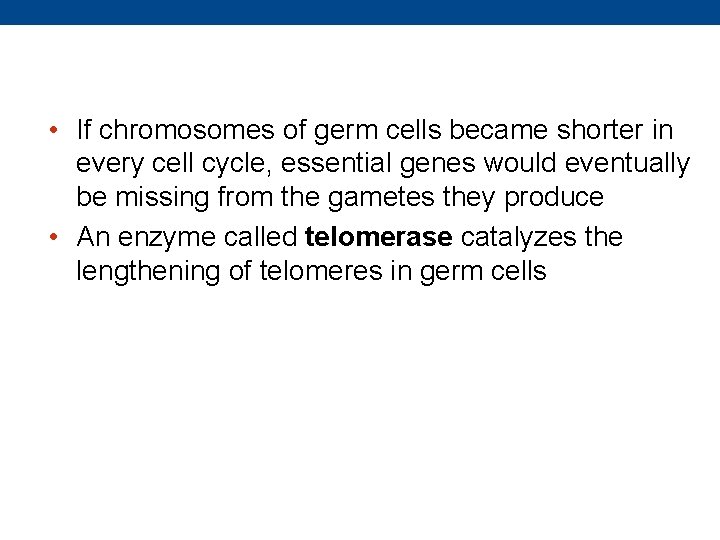
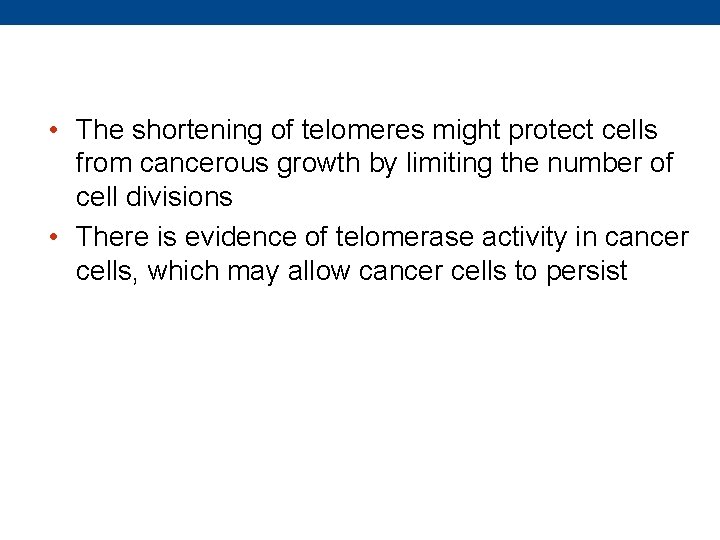
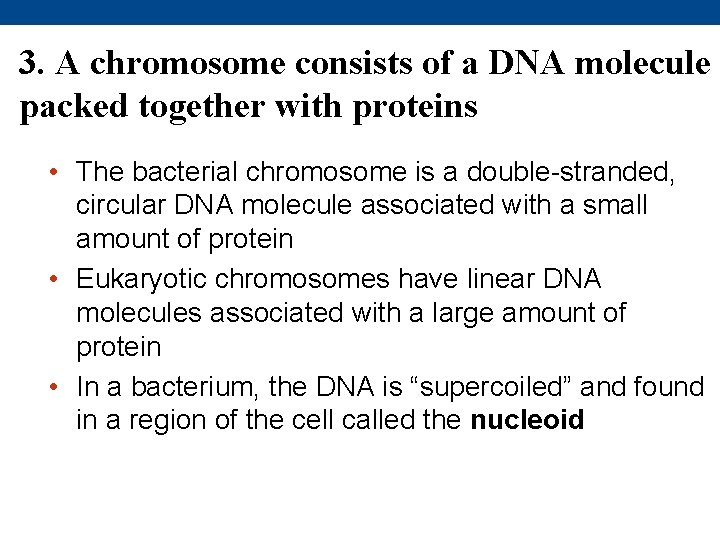
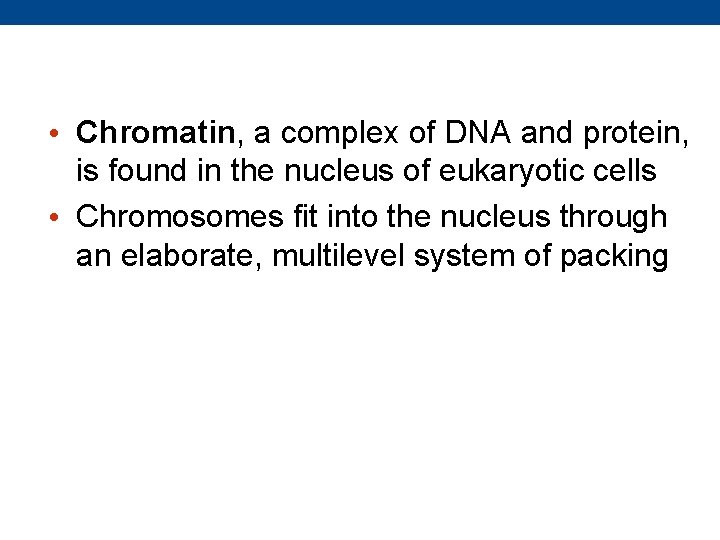
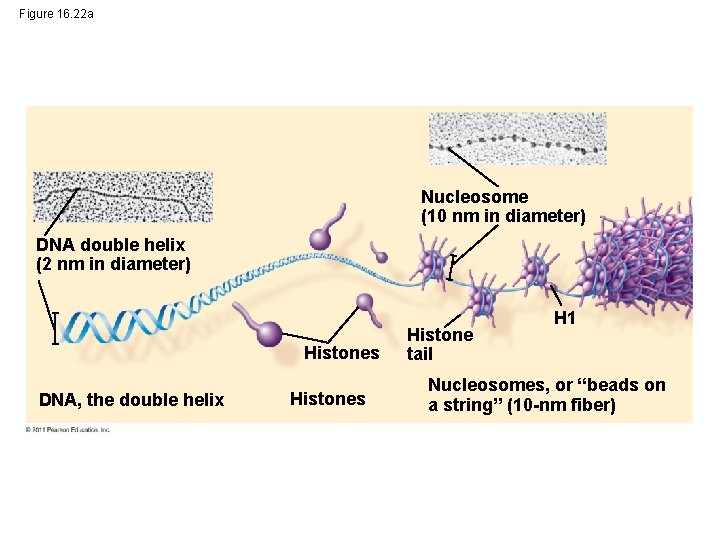
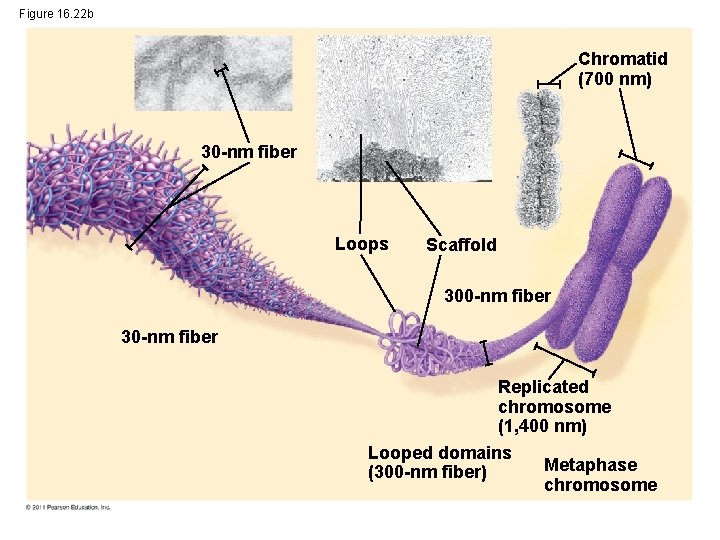
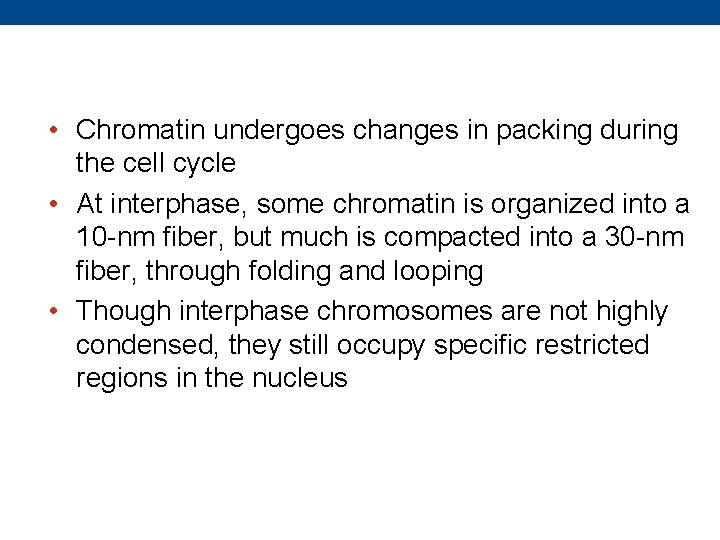
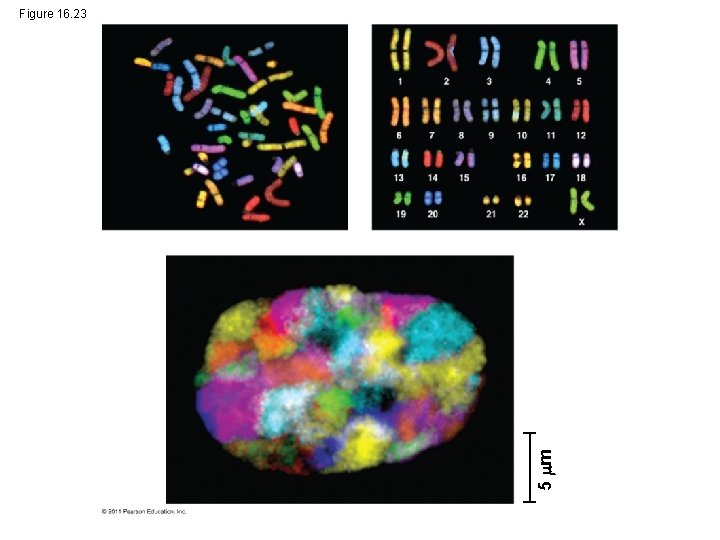
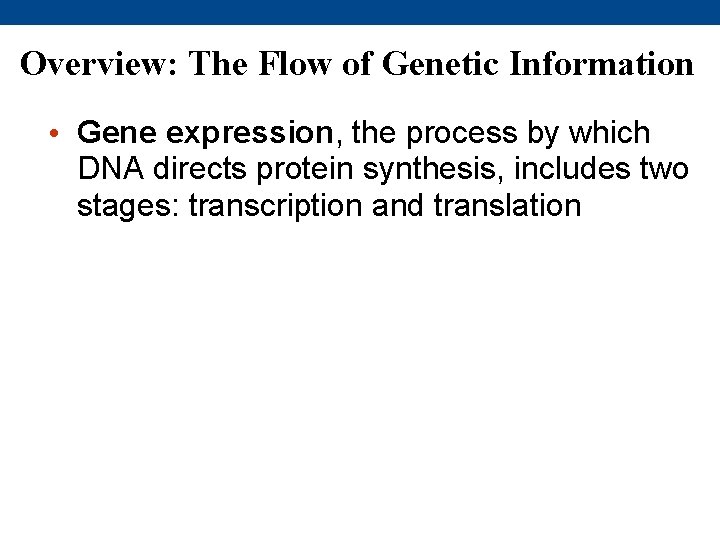
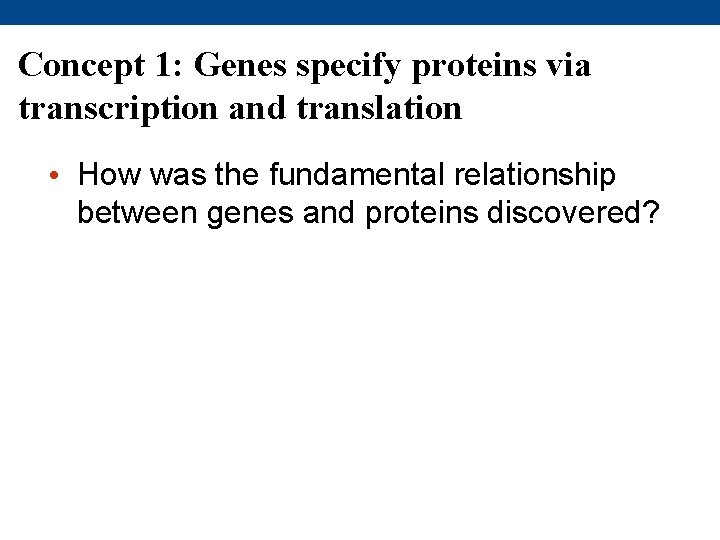
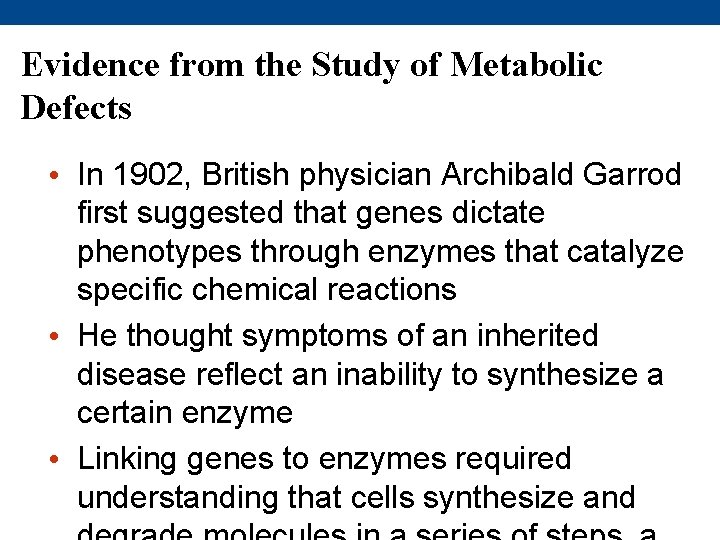
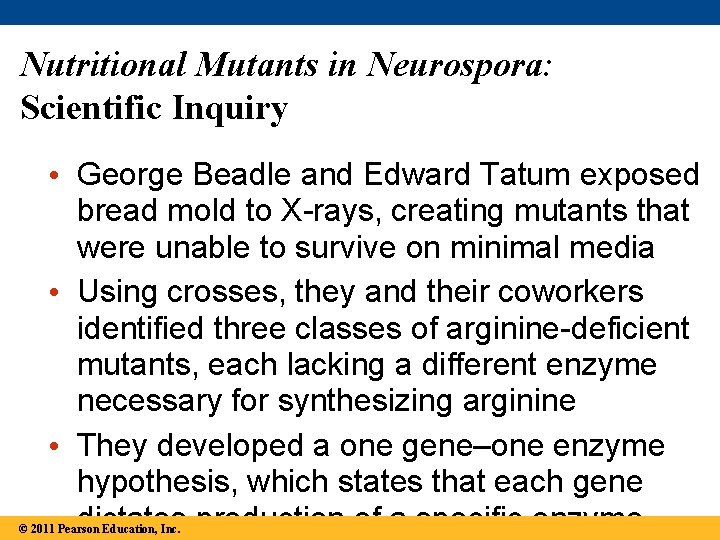
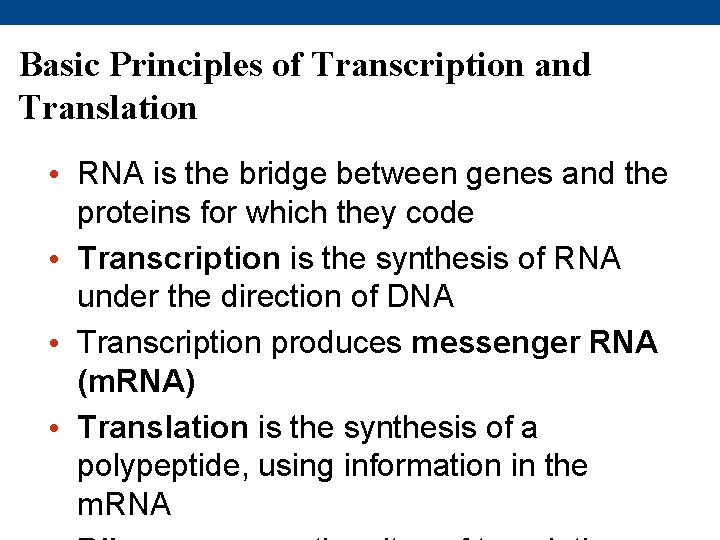
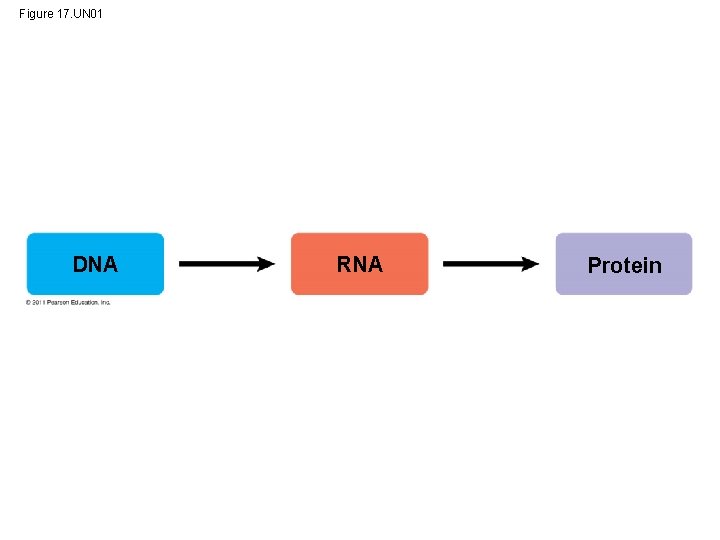
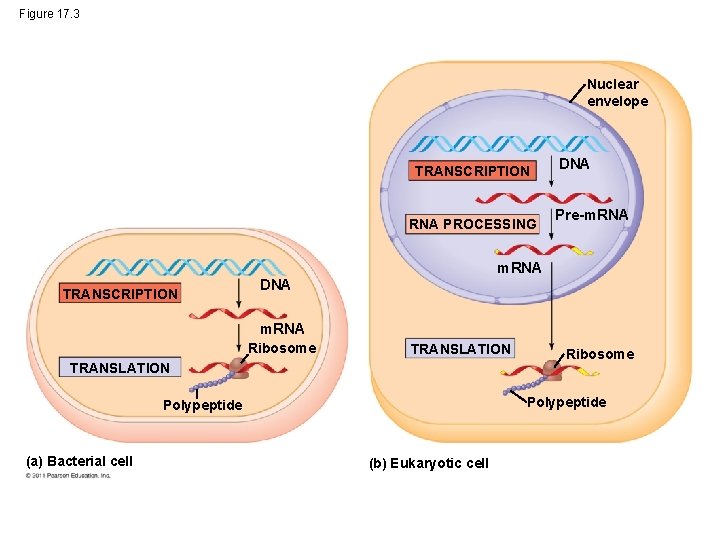
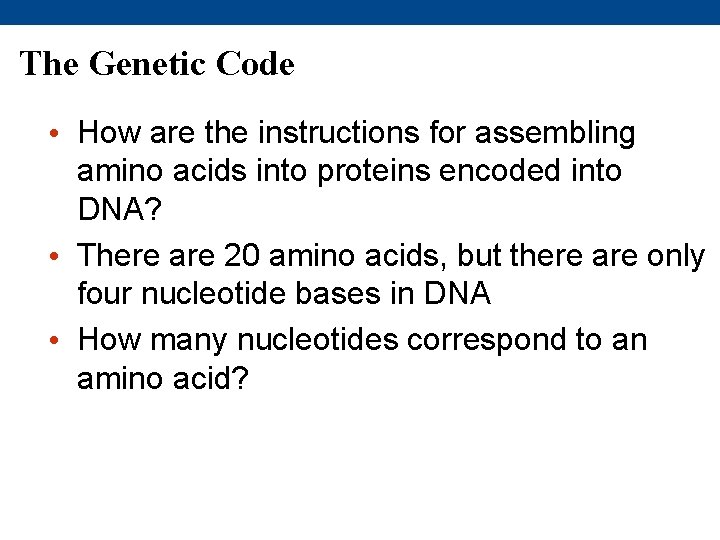
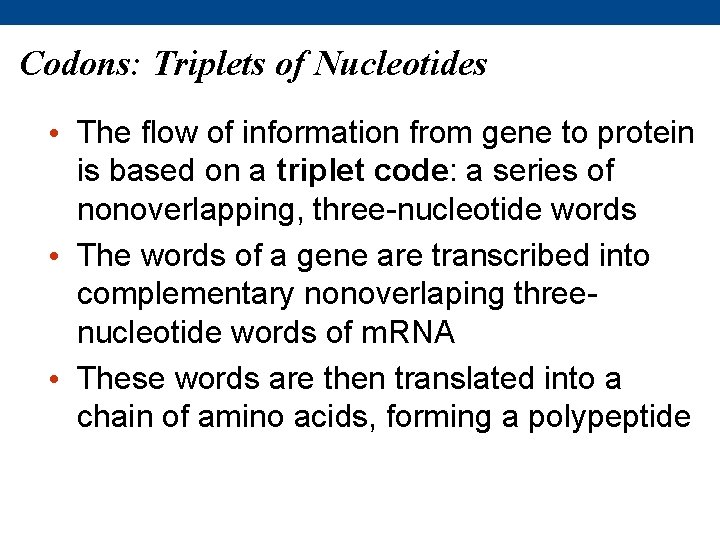
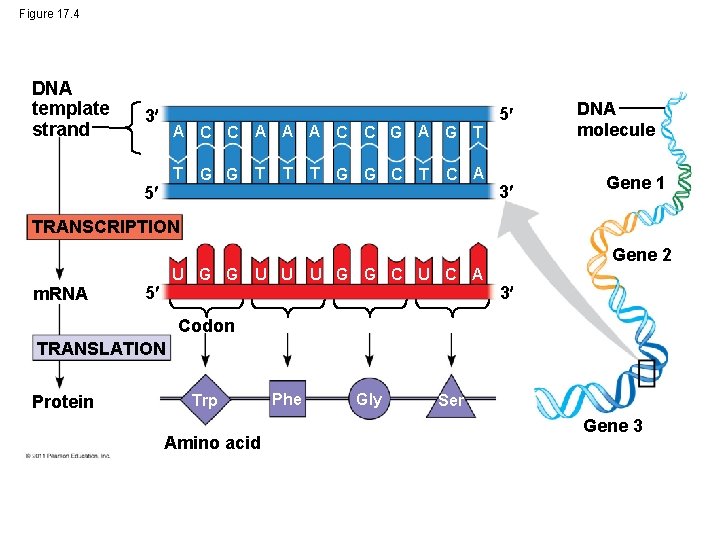
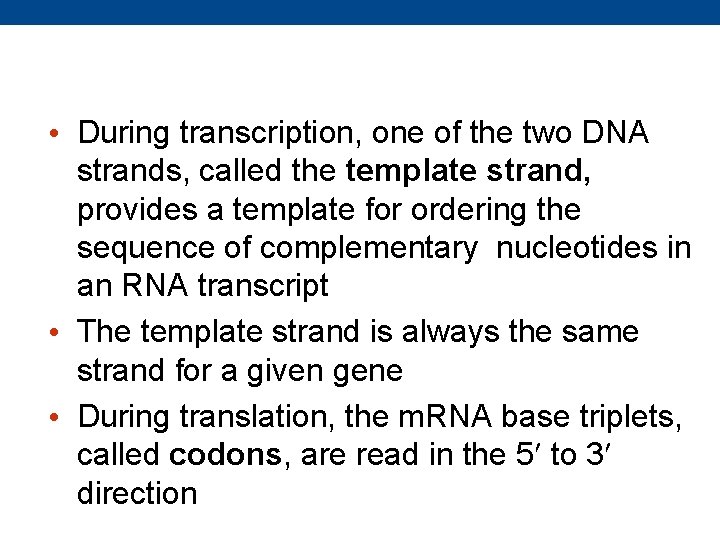
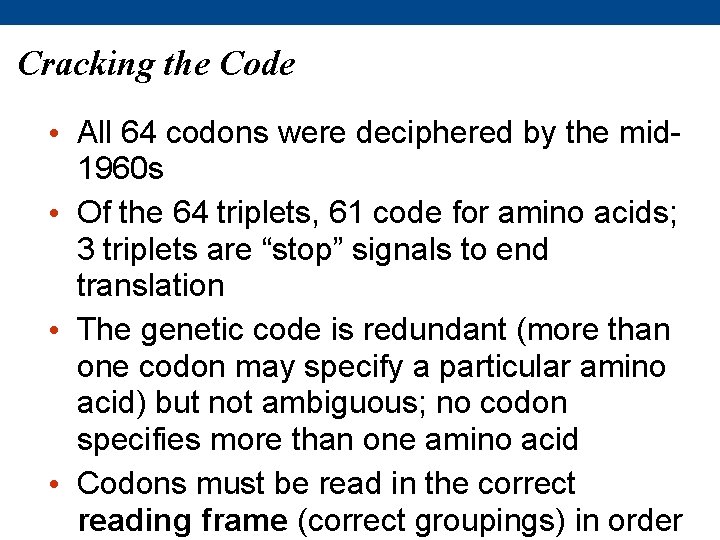
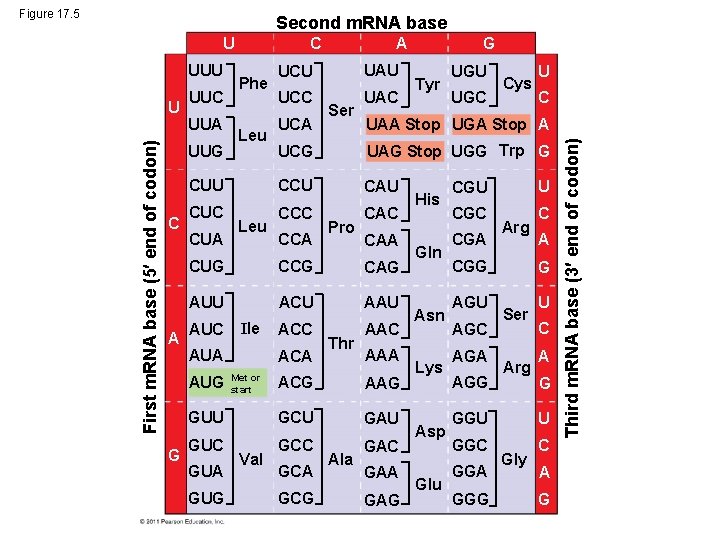
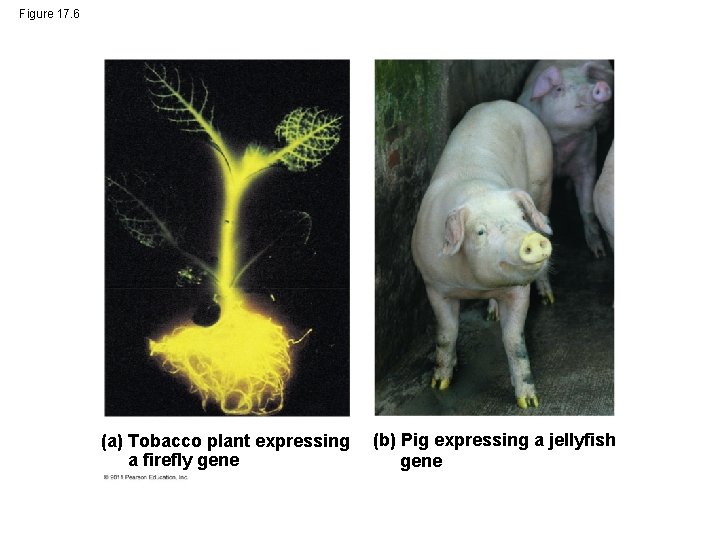
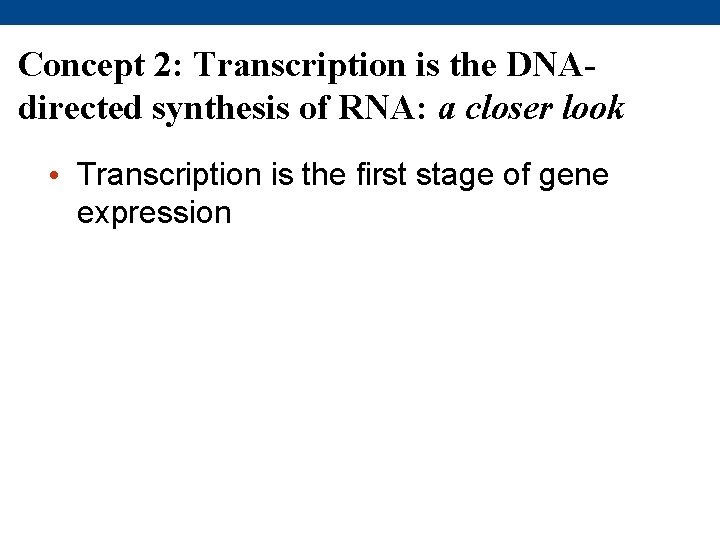
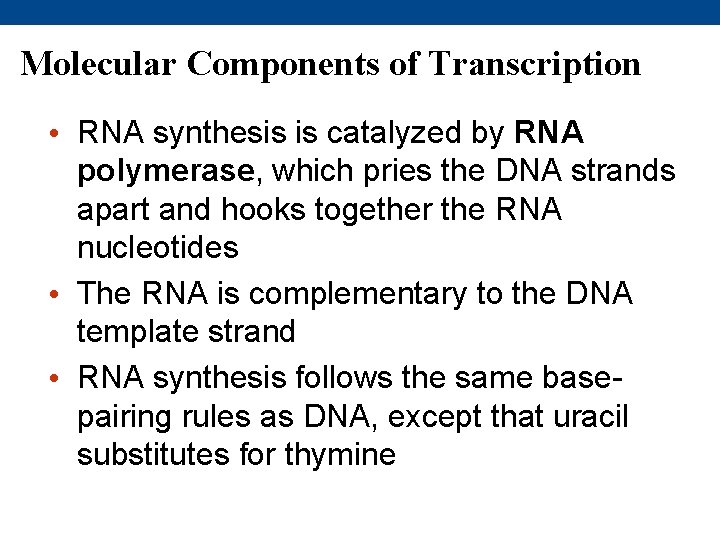
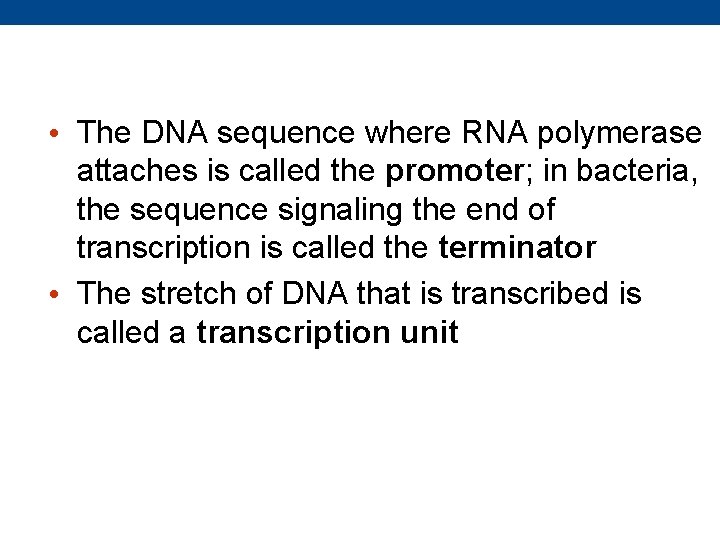
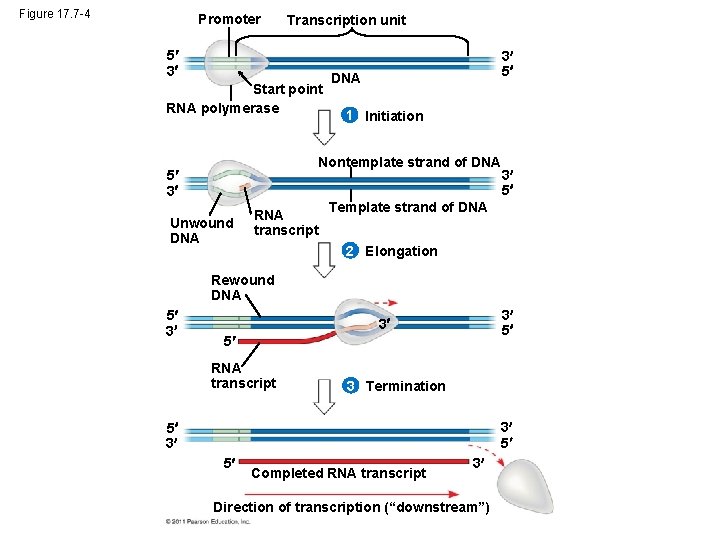
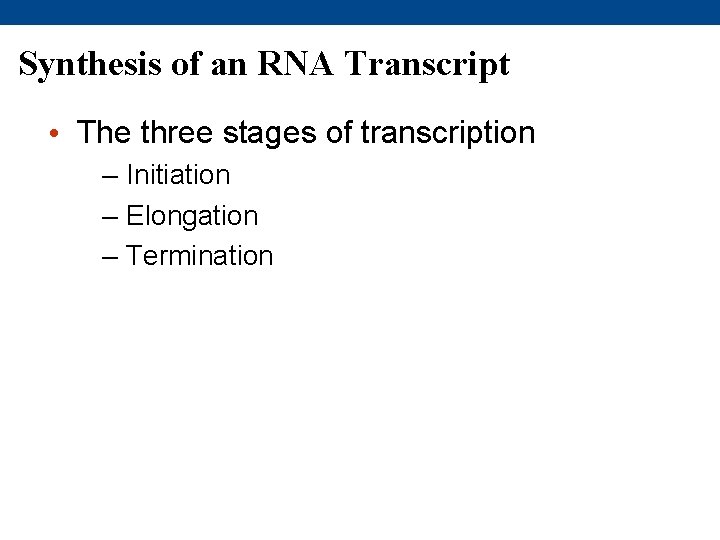
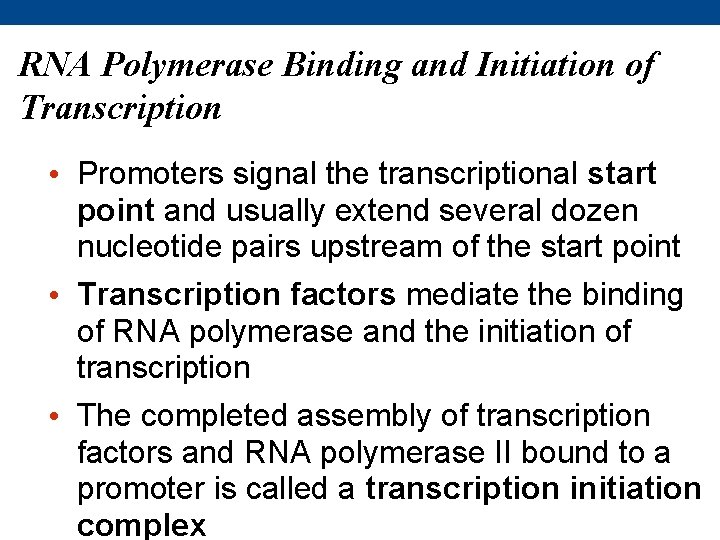
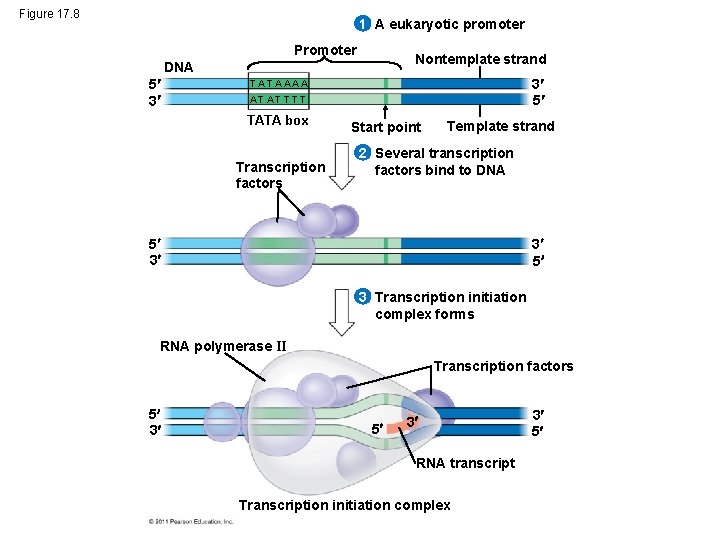
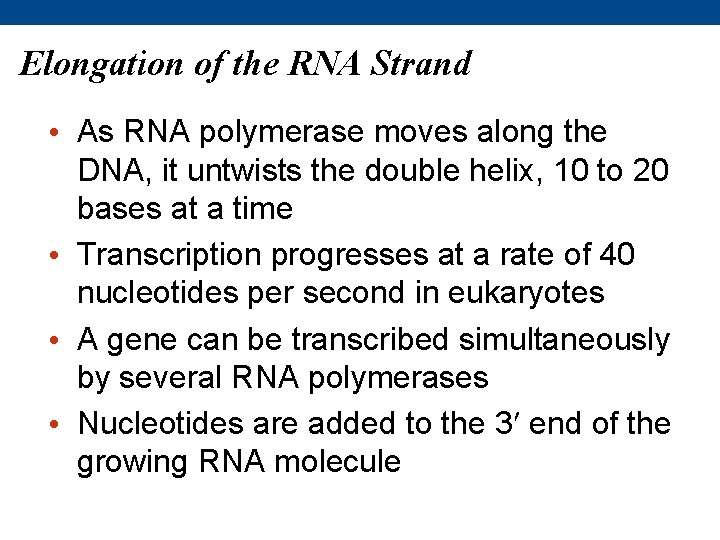
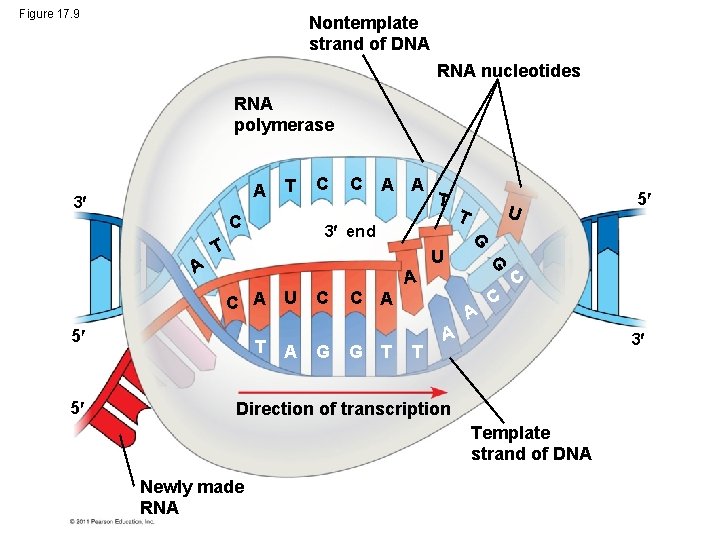
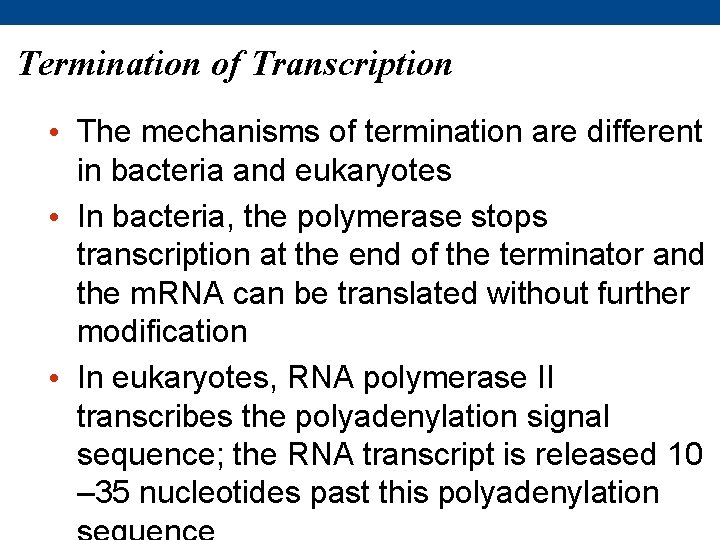
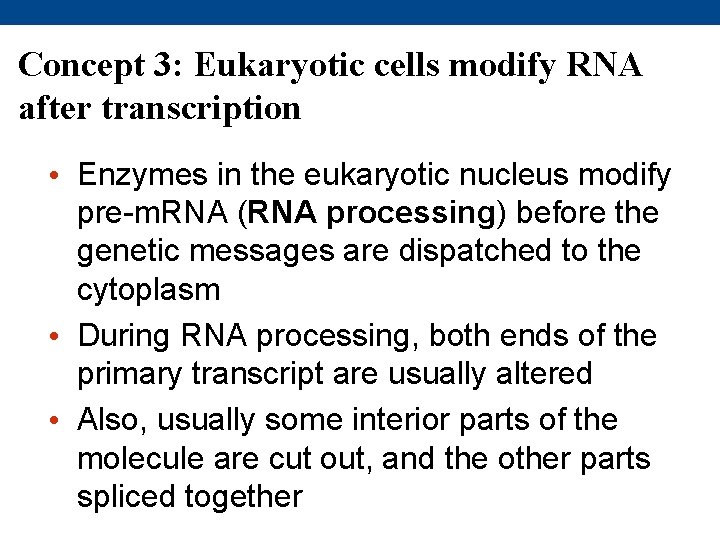
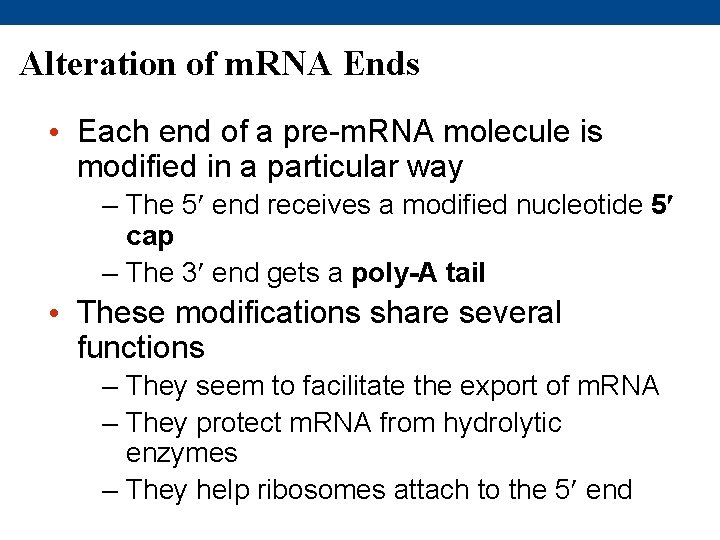
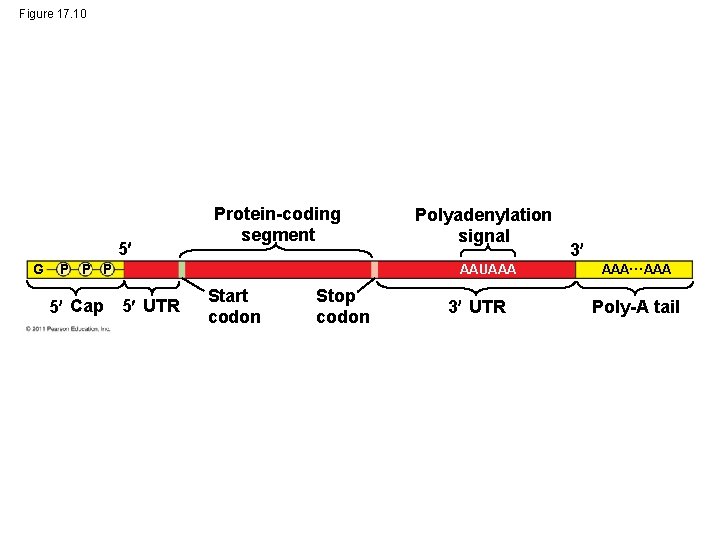
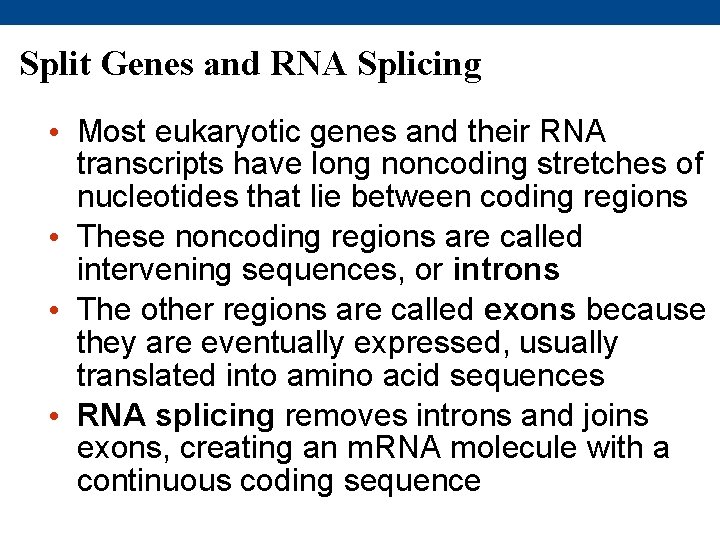
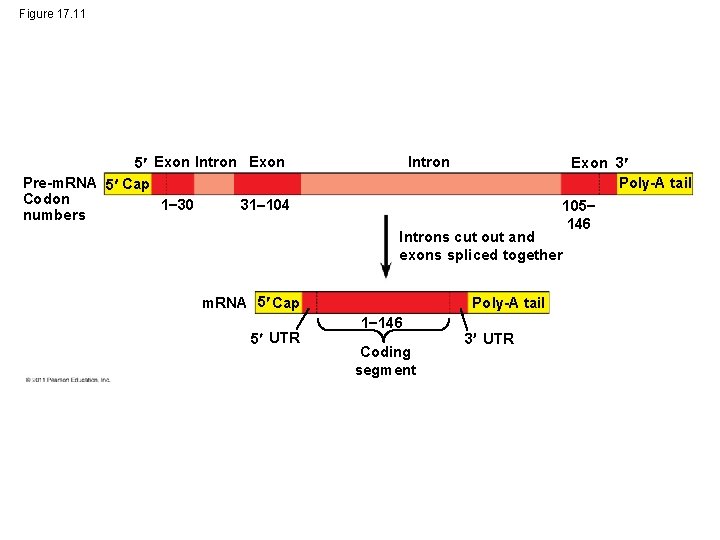
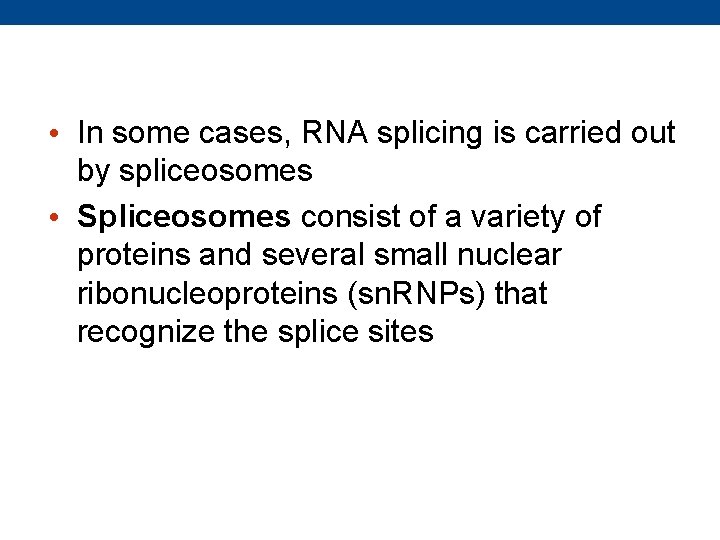
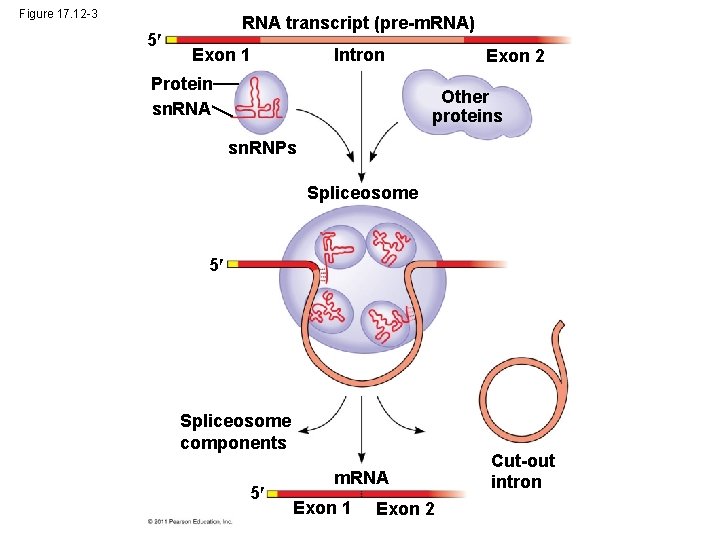
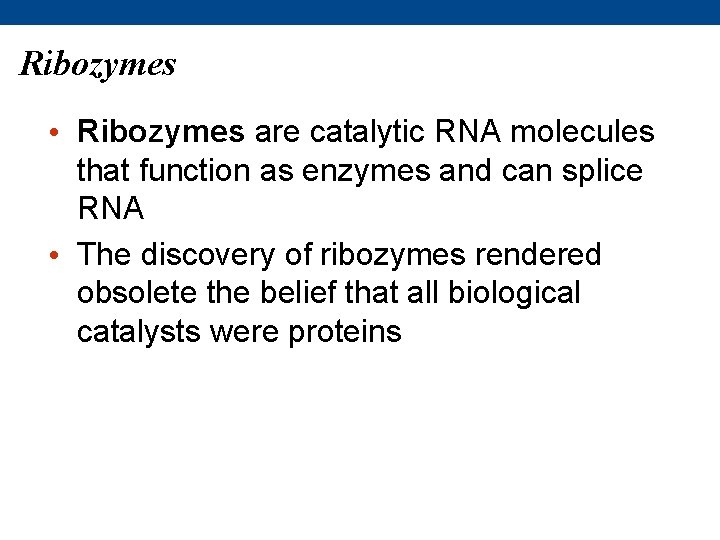
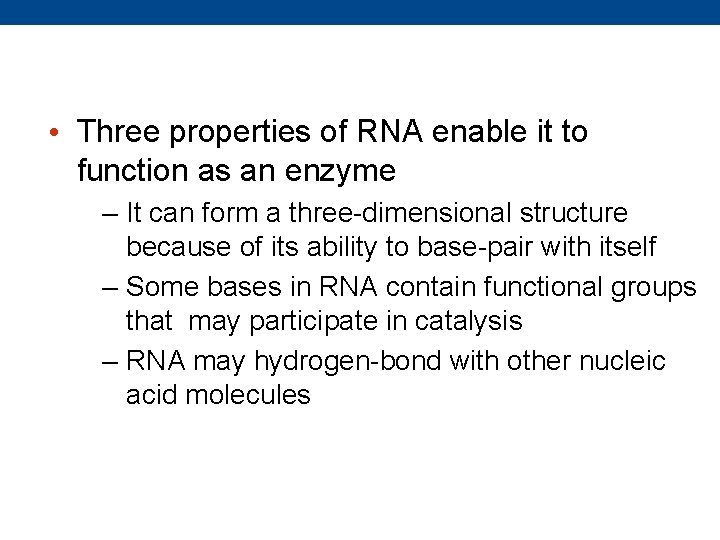
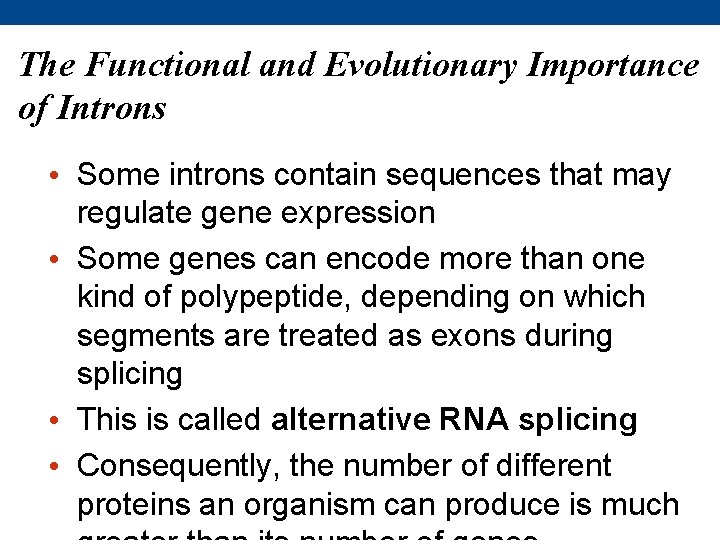
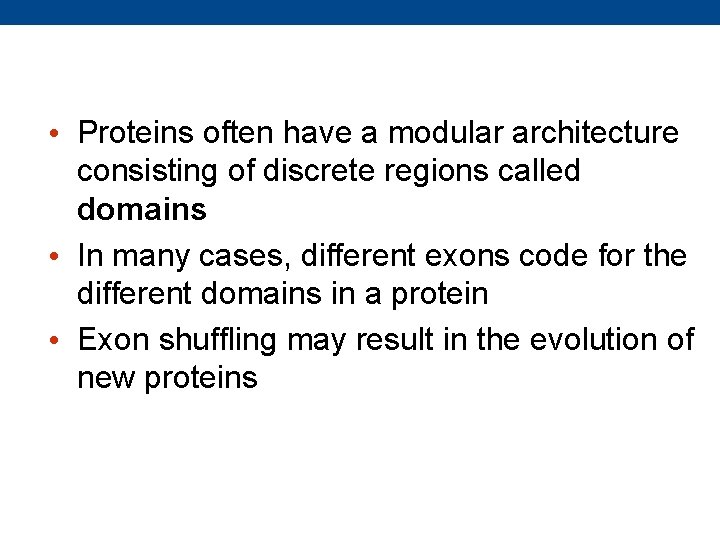
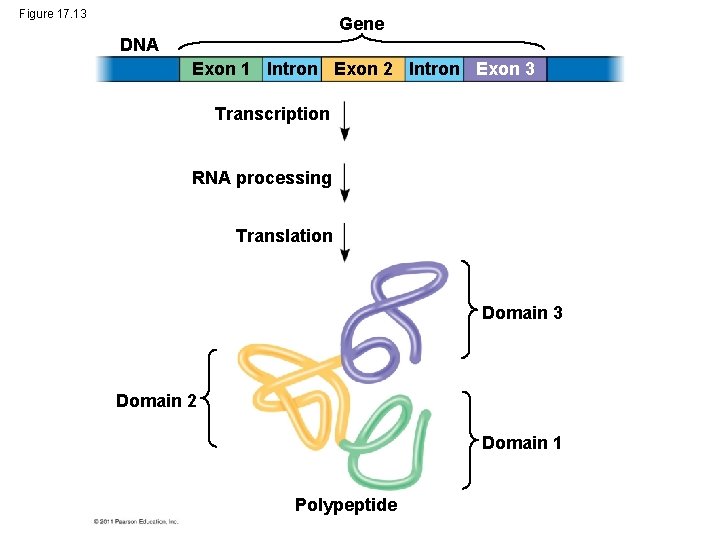
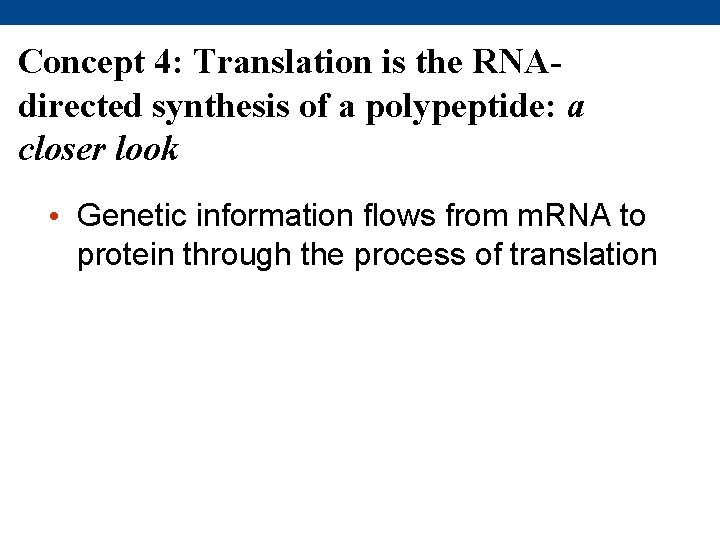
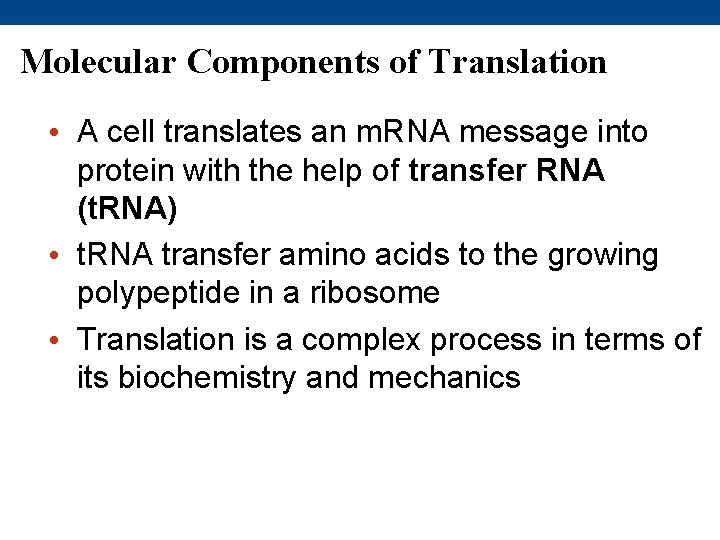
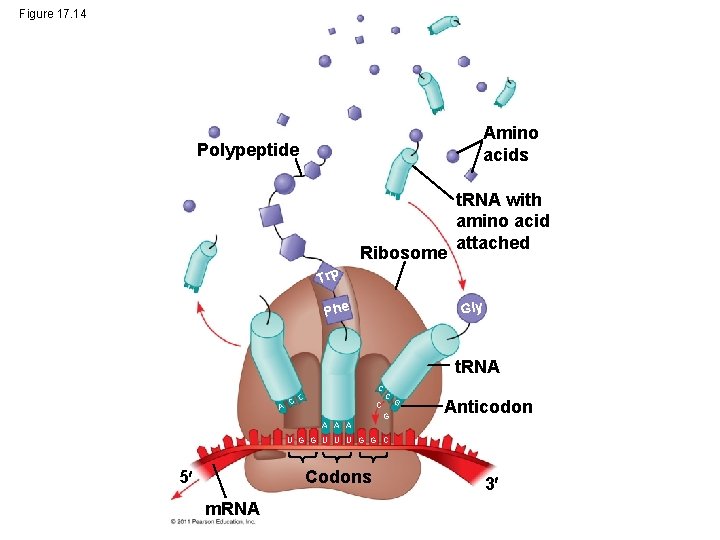
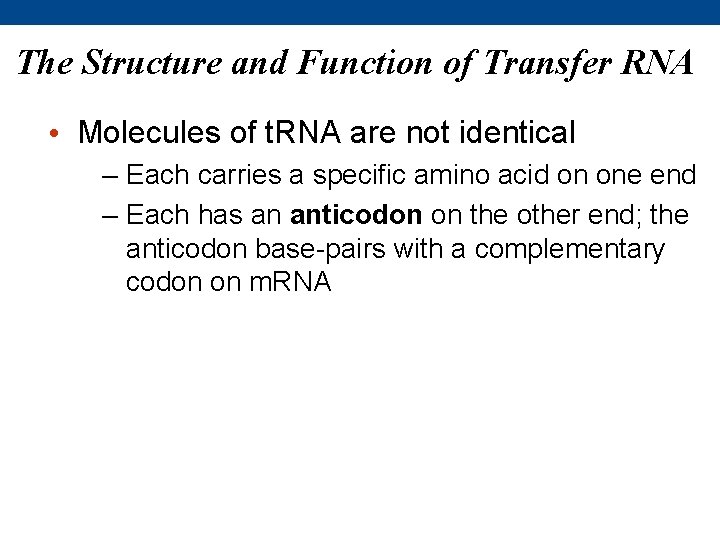
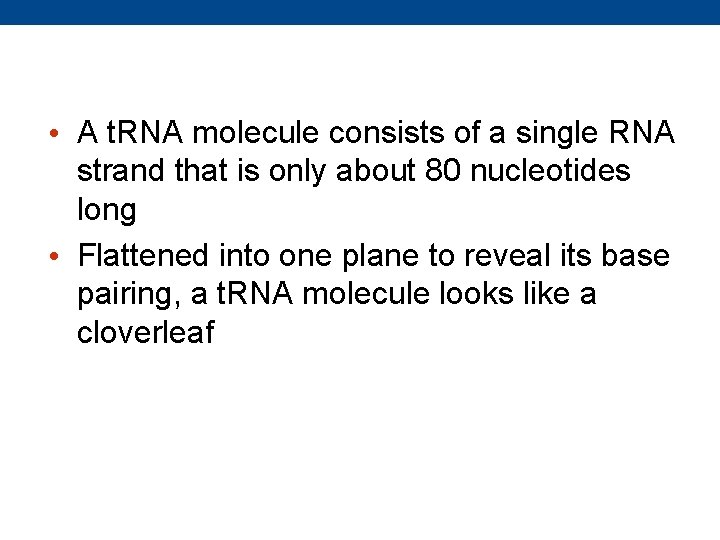
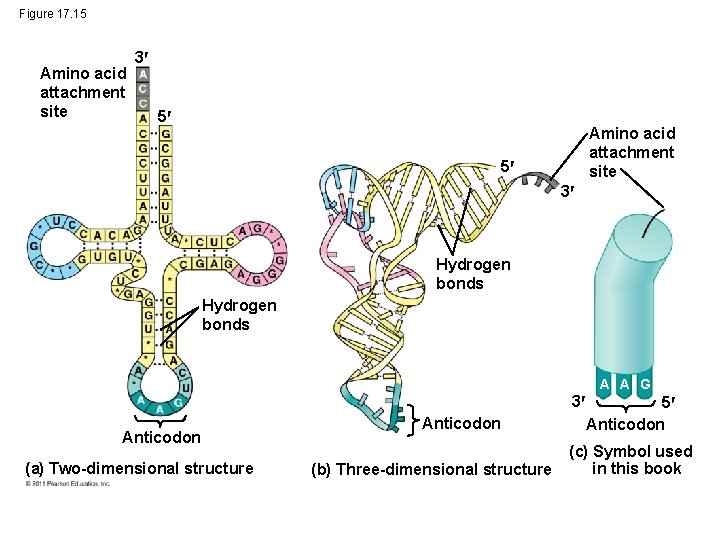
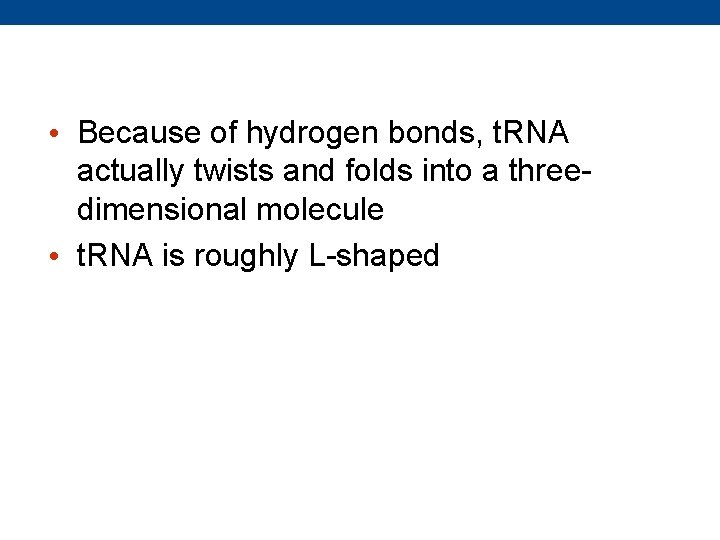
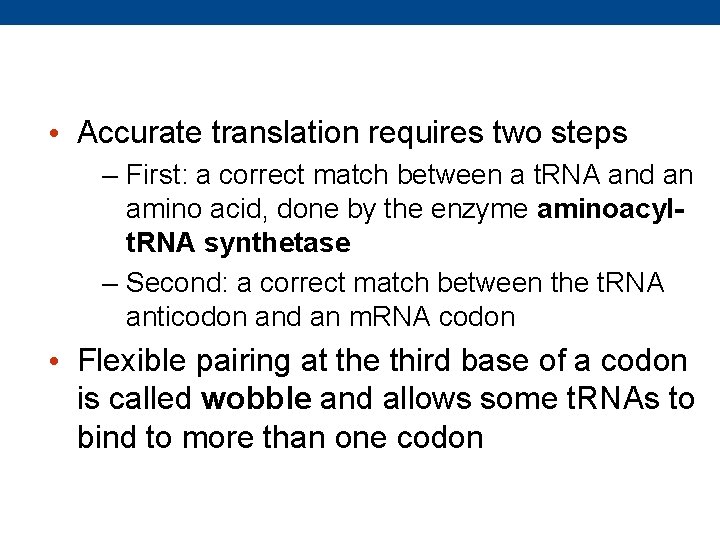
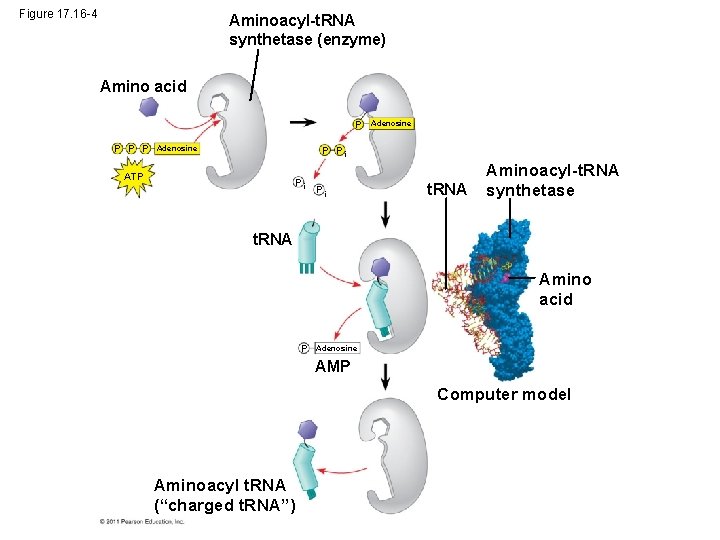
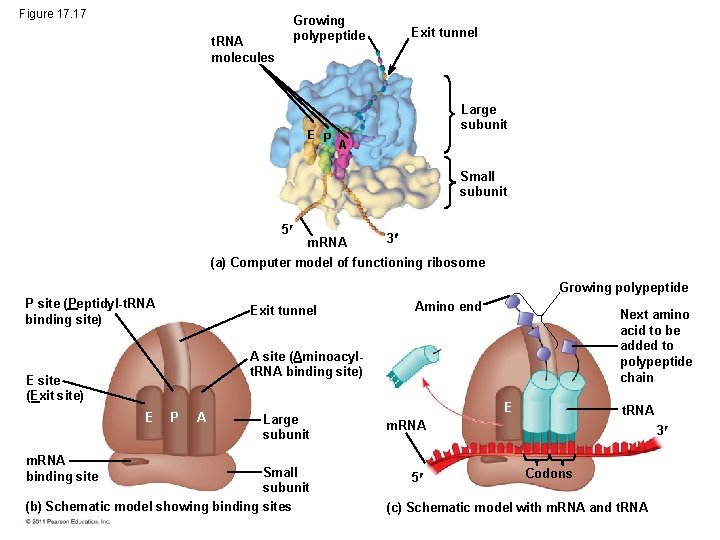
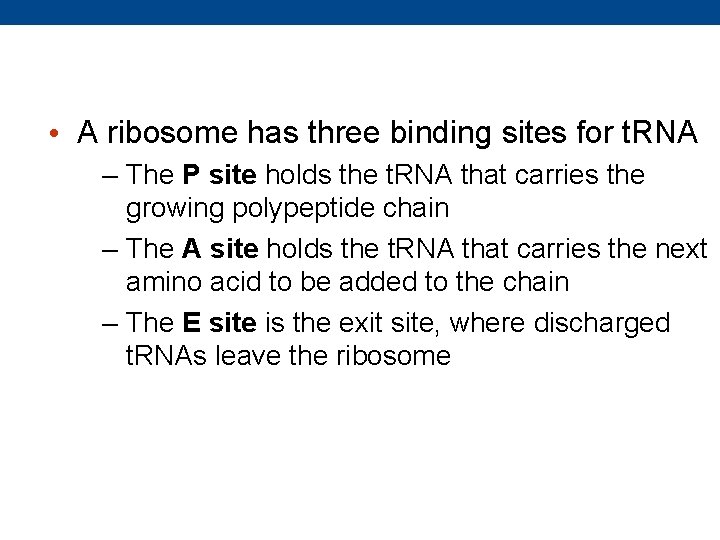
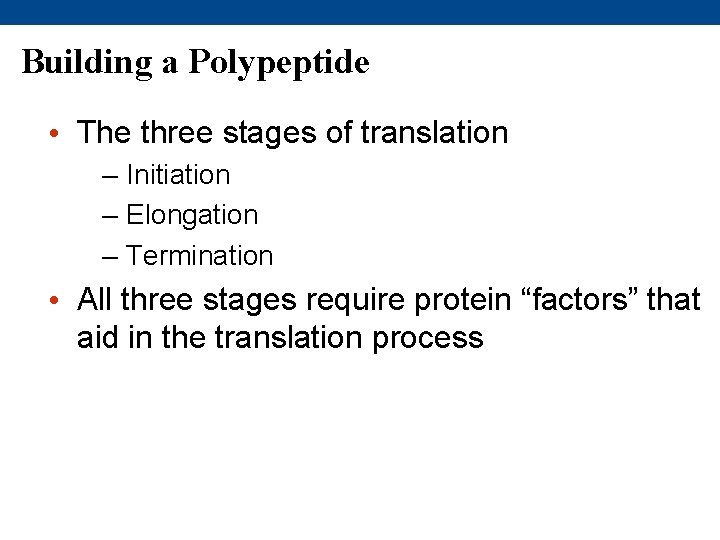
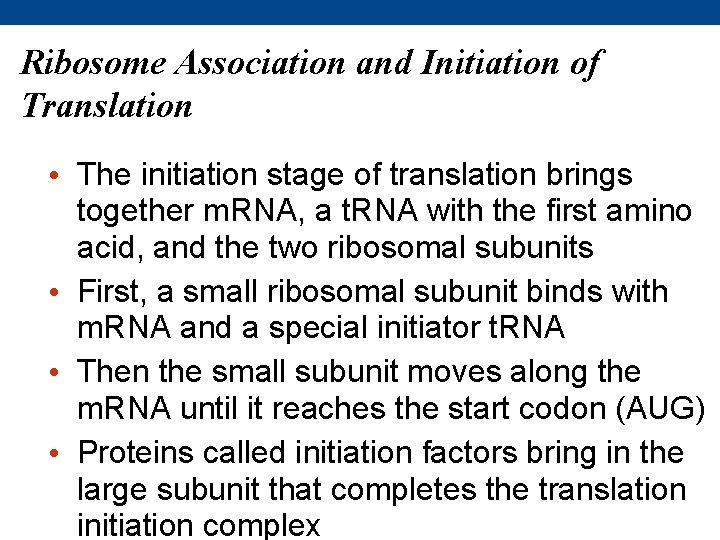
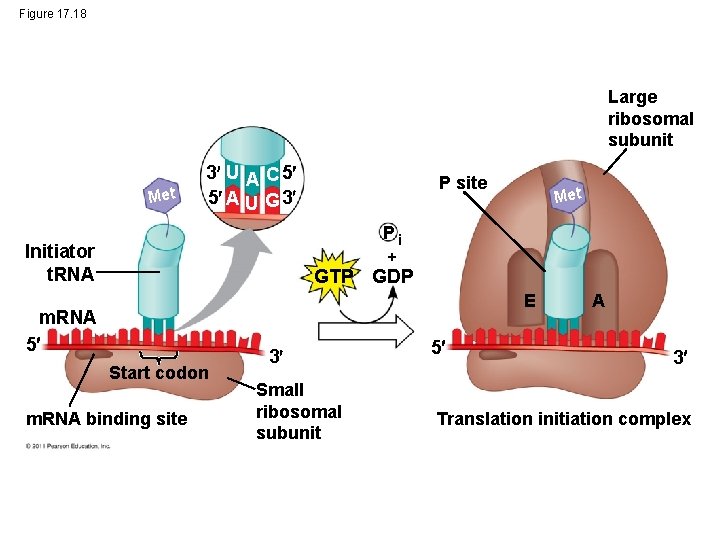
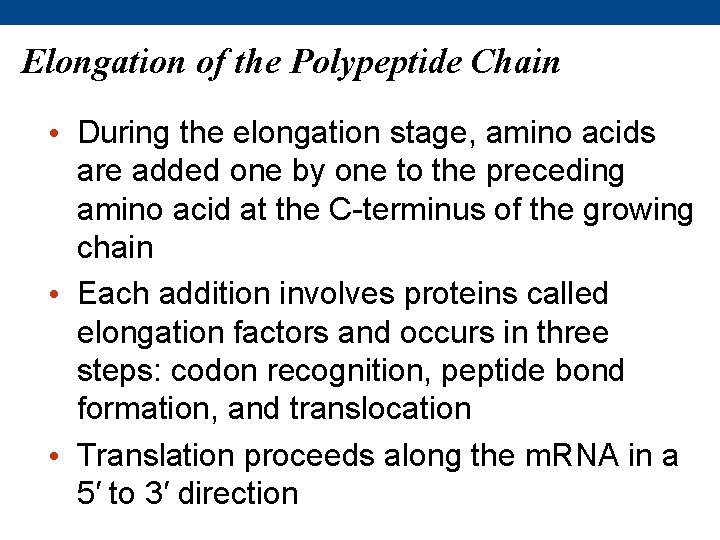
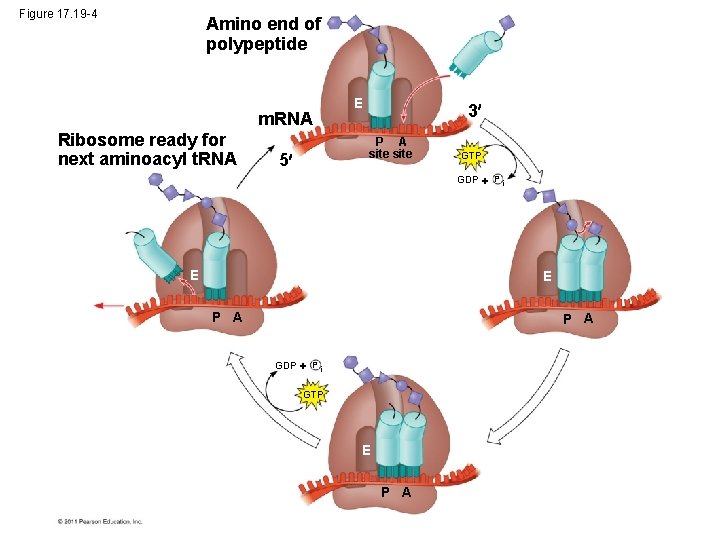
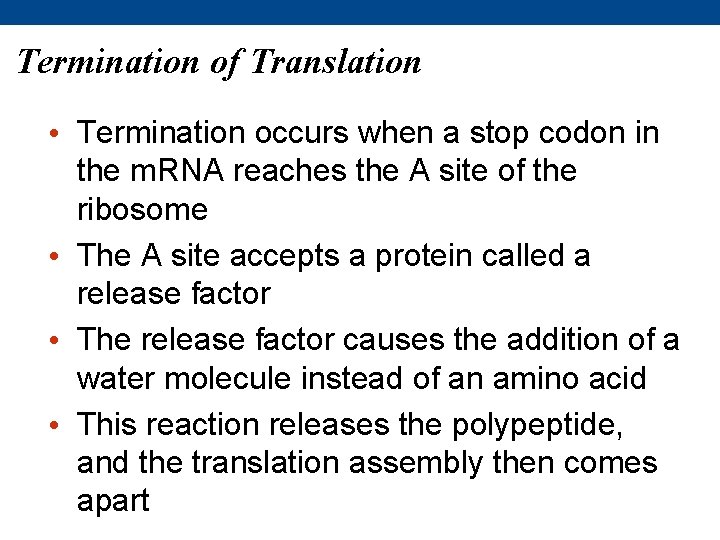
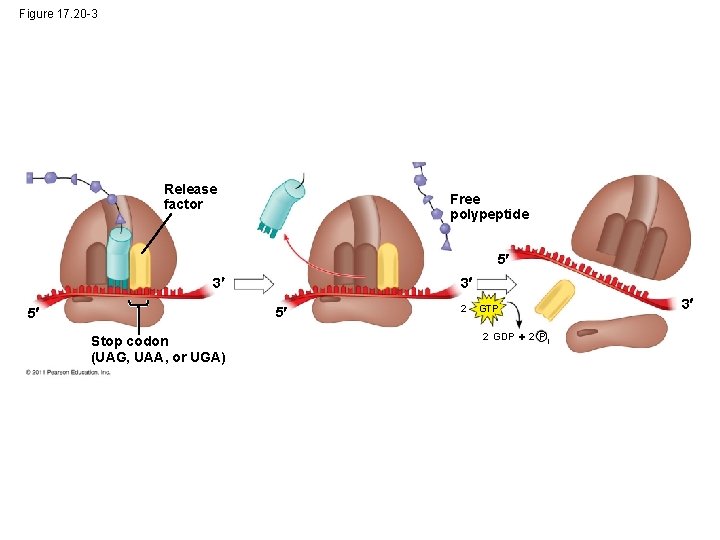
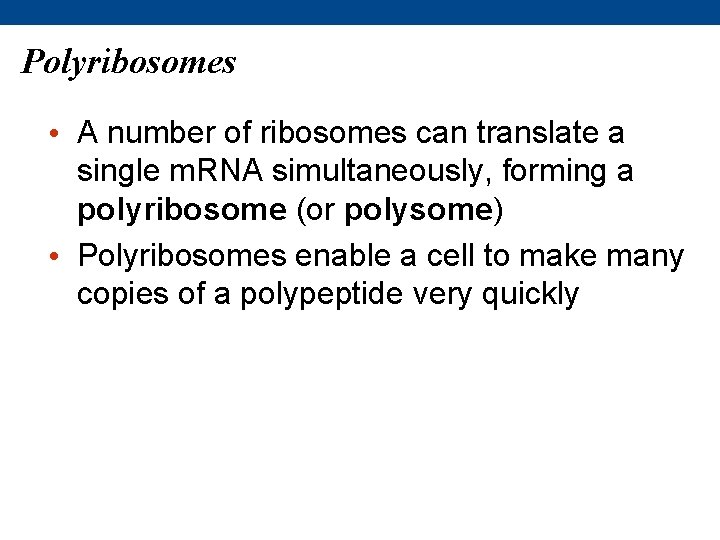
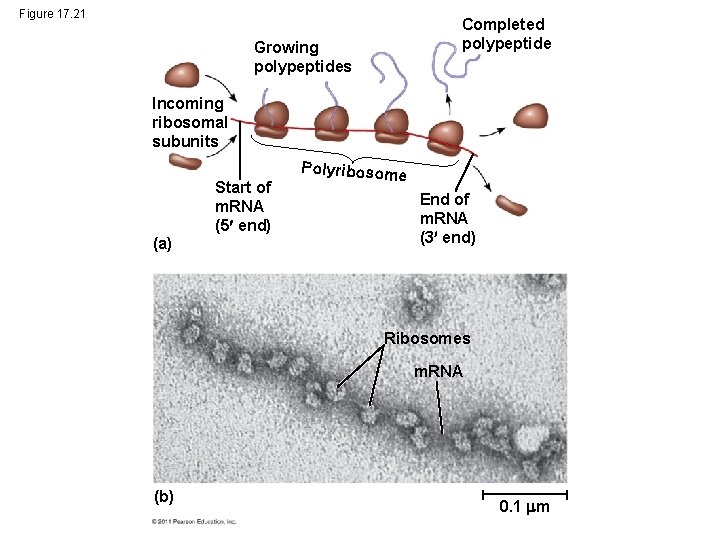
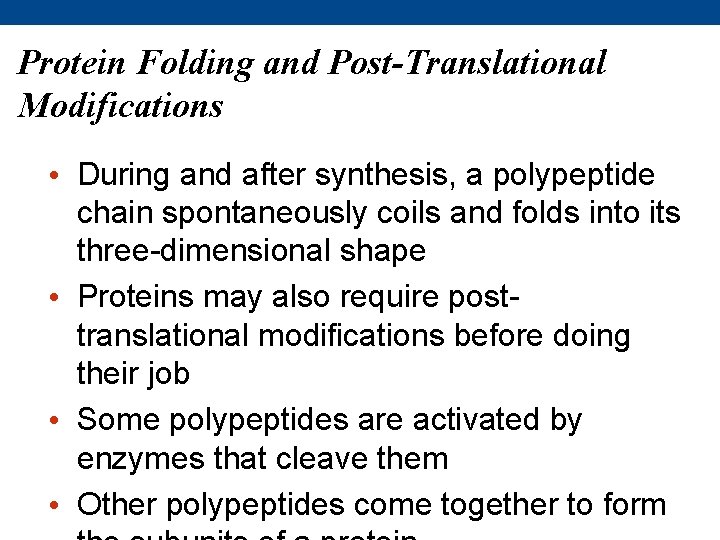
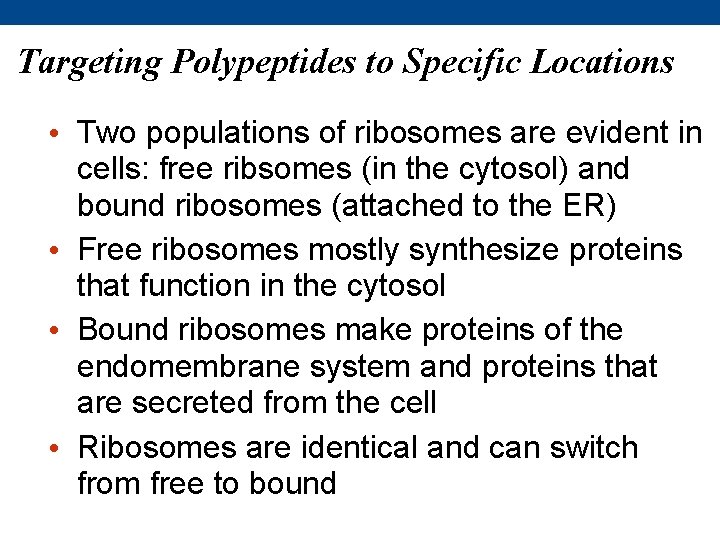
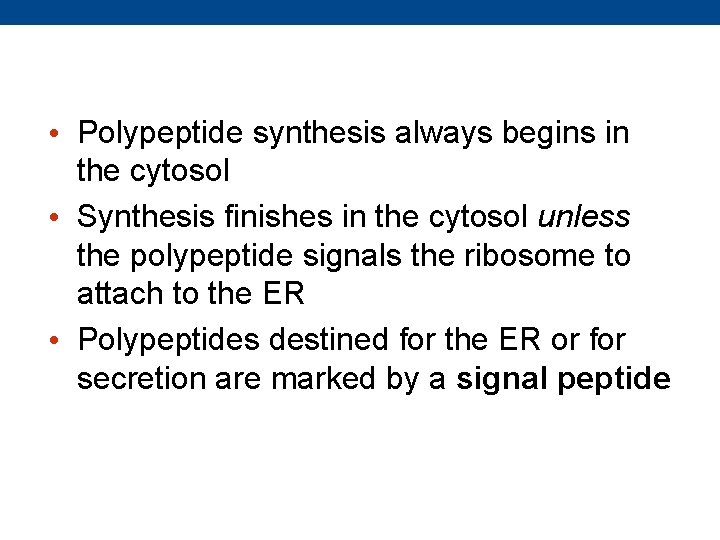
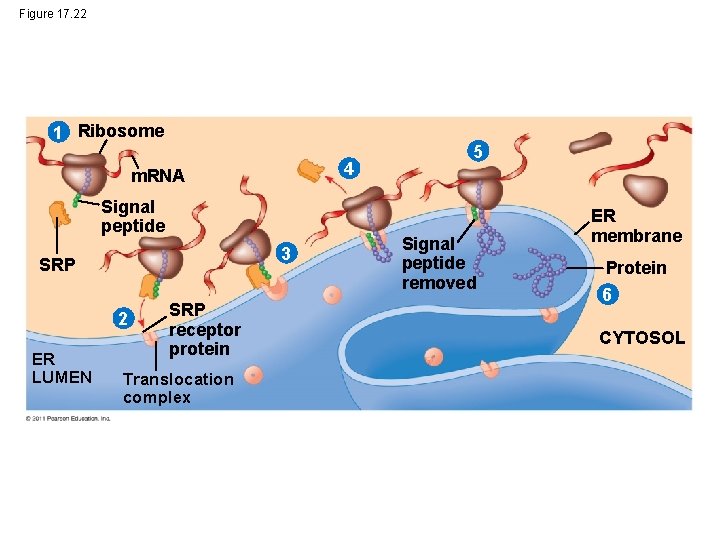
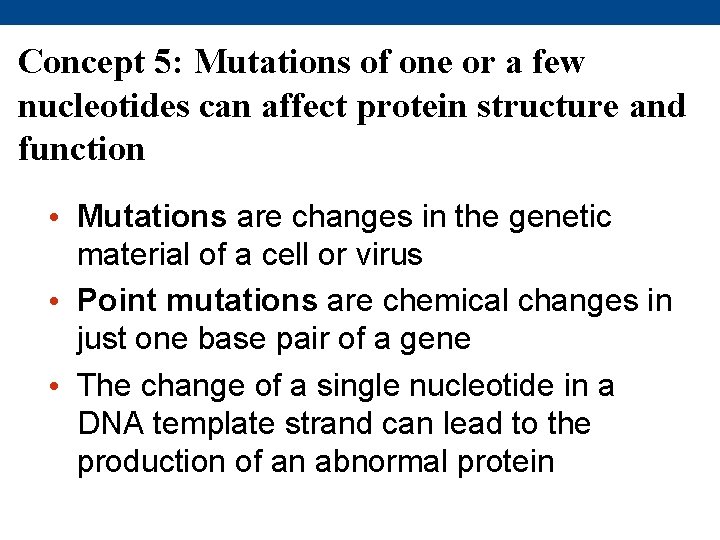
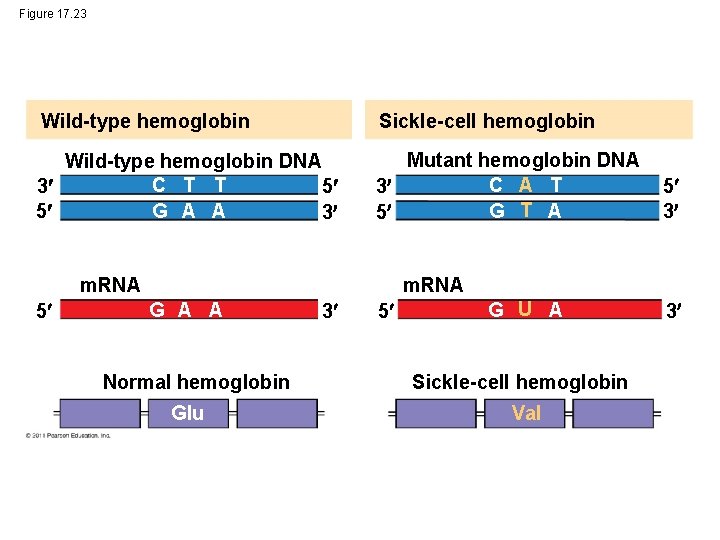
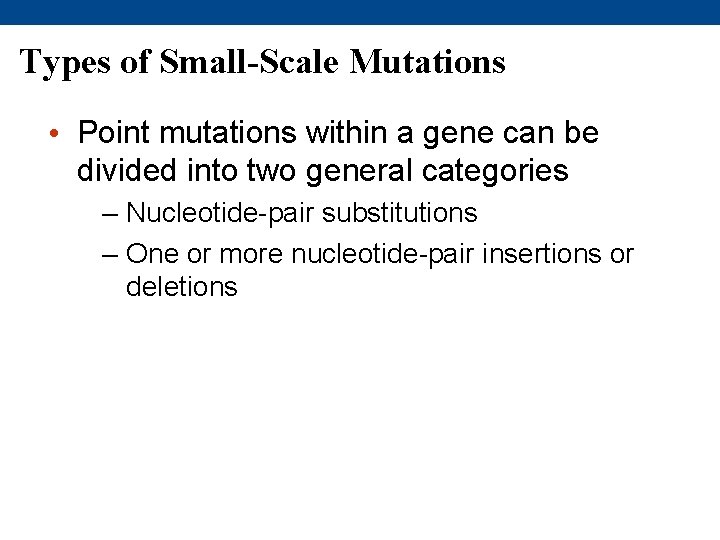
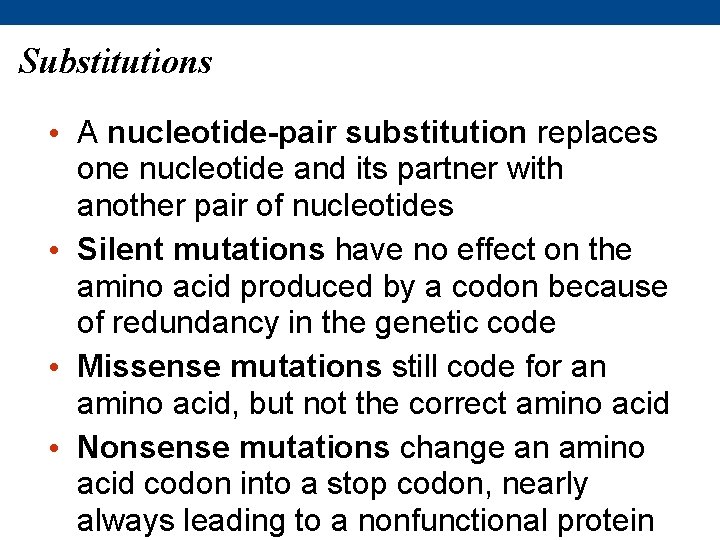
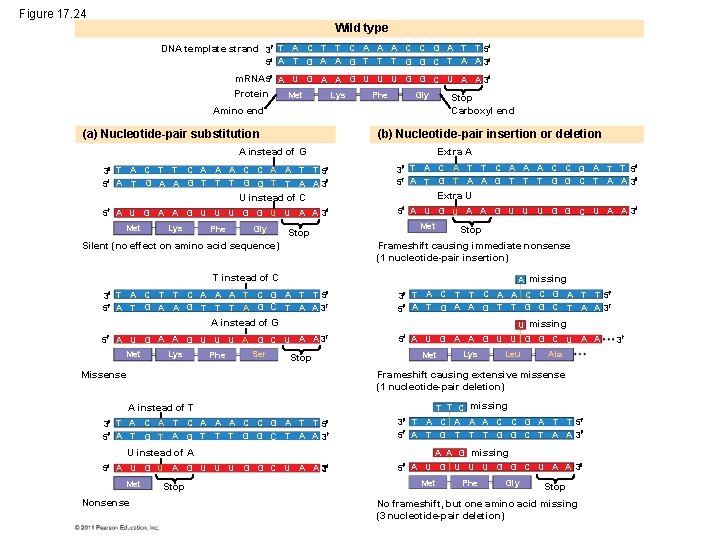
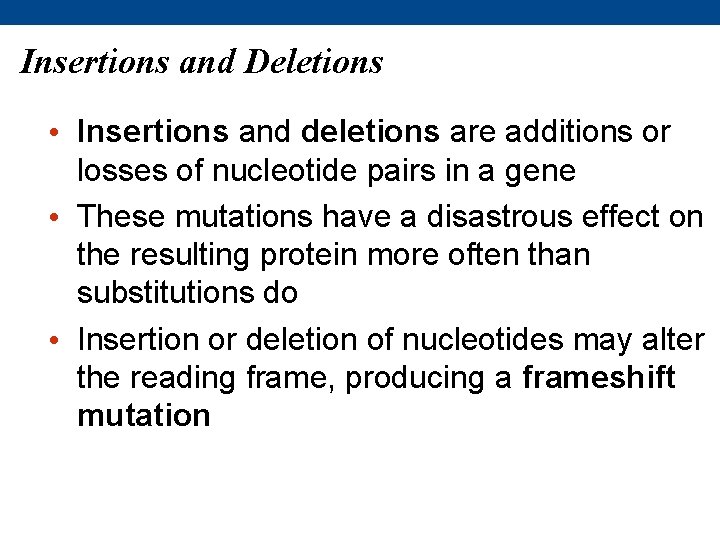
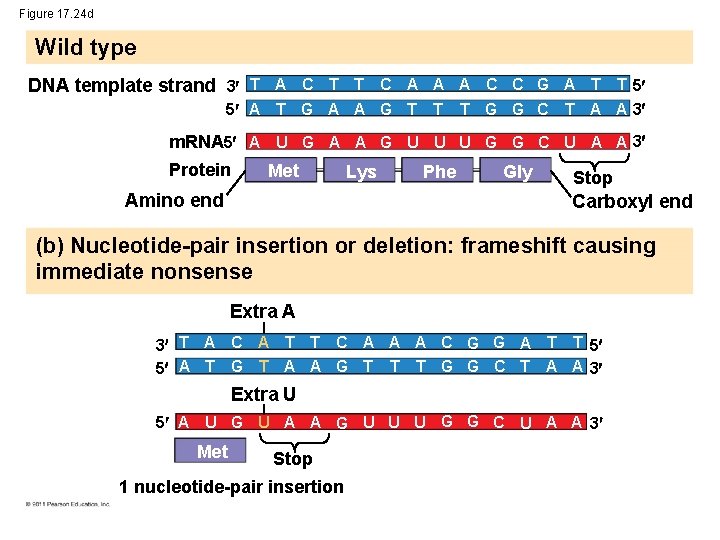
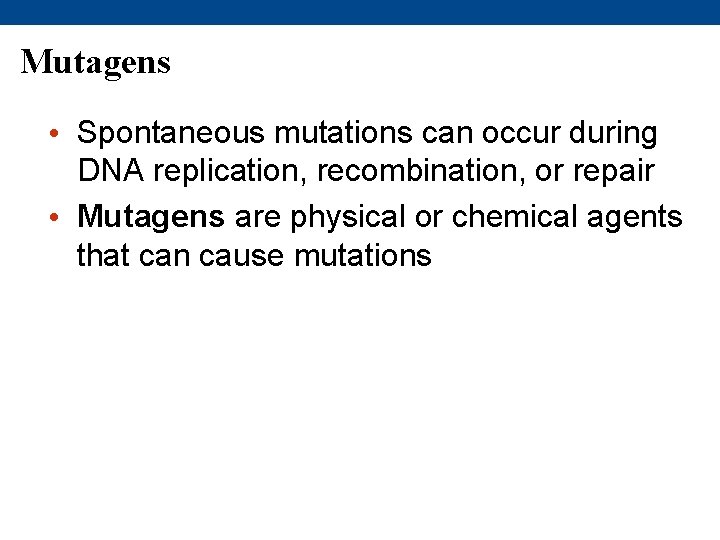
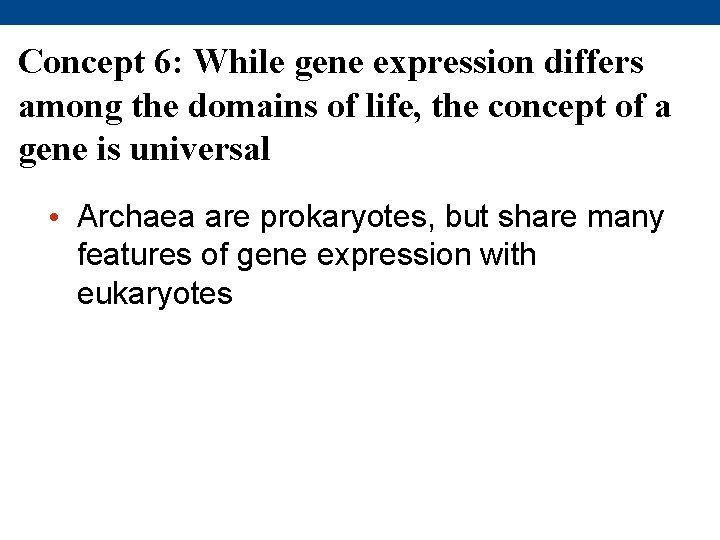
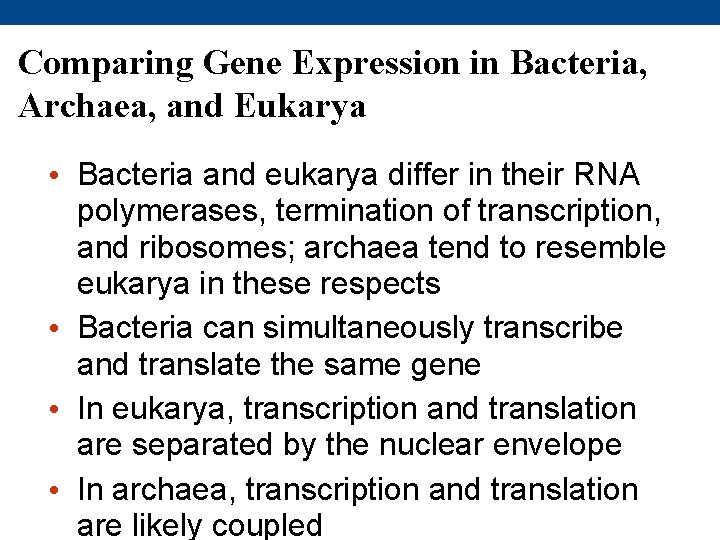
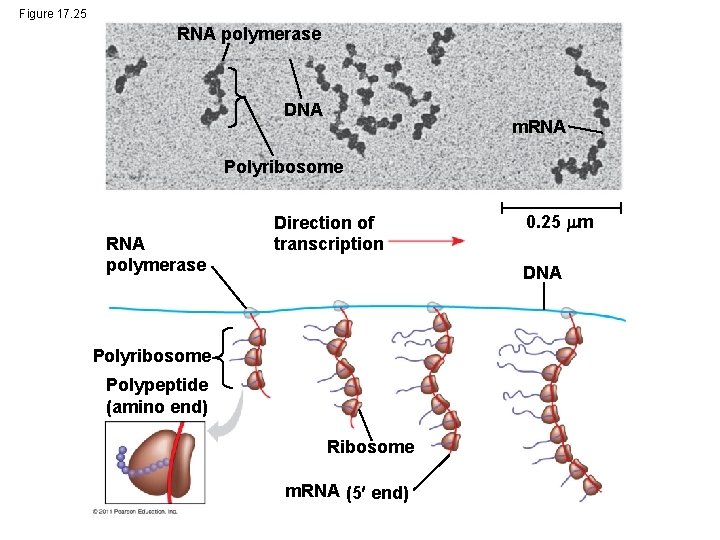
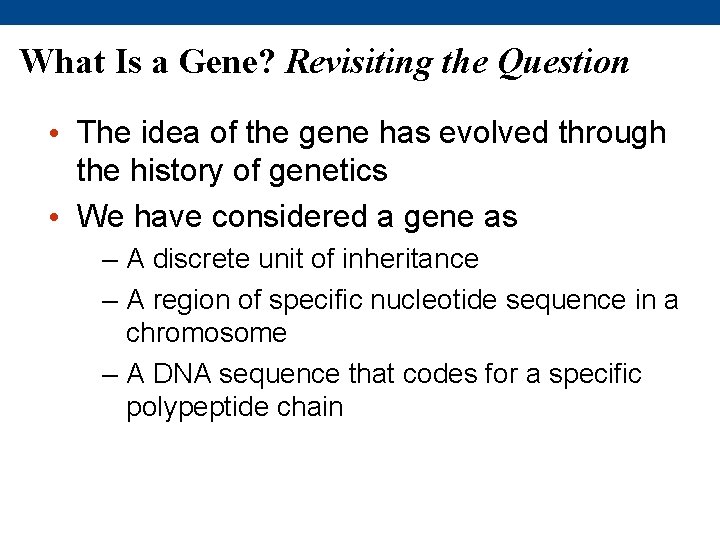
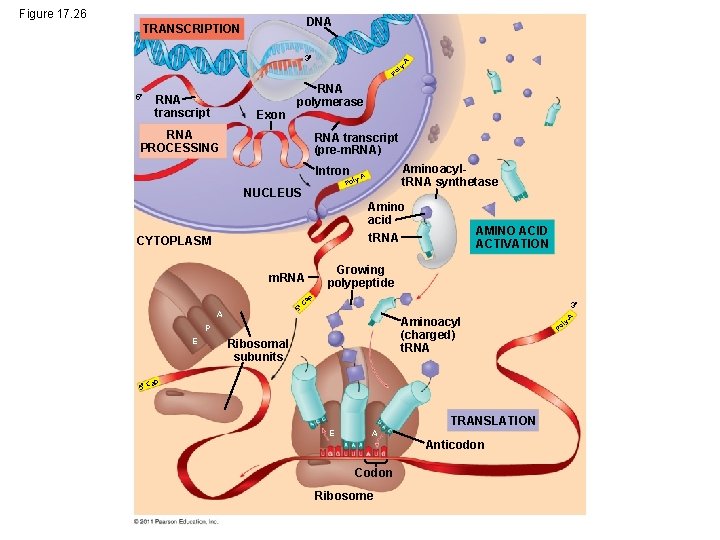
- Slides: 127
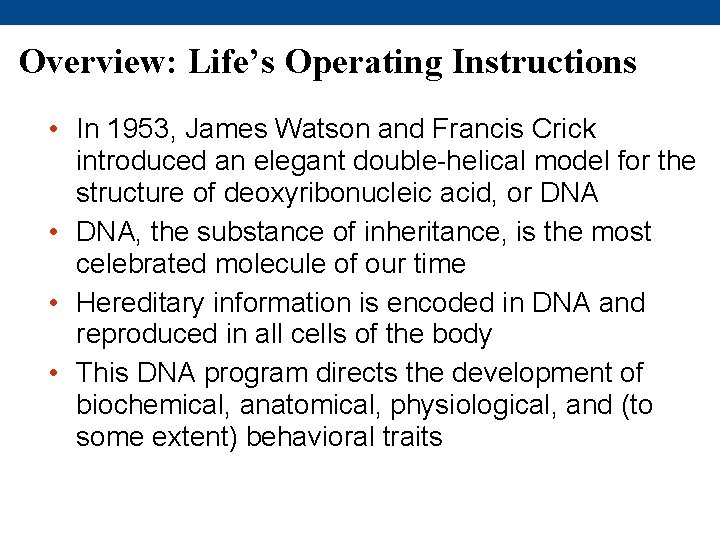
Overview: Life’s Operating Instructions • In 1953, James Watson and Francis Crick introduced an elegant double-helical model for the structure of deoxyribonucleic acid, or DNA • DNA, the substance of inheritance, is the most celebrated molecule of our time • Hereditary information is encoded in DNA and reproduced in all cells of the body • This DNA program directs the development of biochemical, anatomical, physiological, and (to some extent) behavioral traits
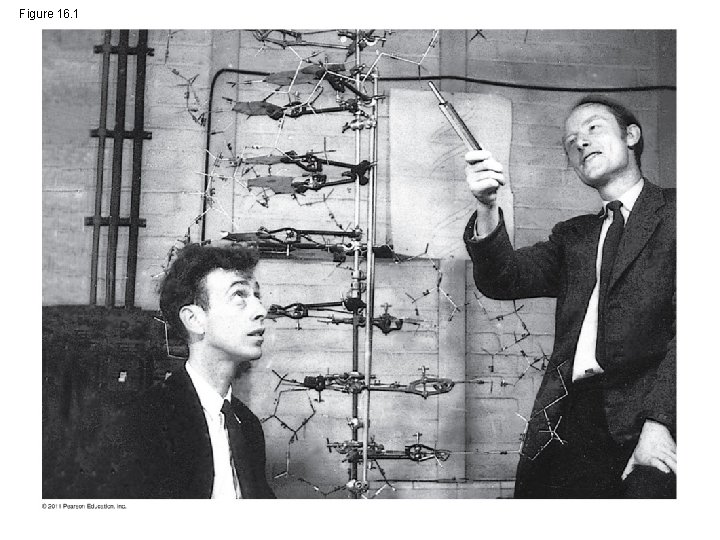
Figure 16. 1
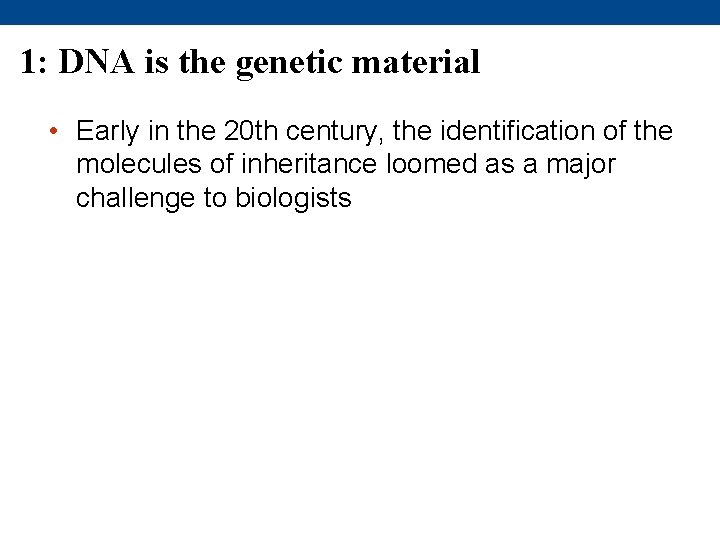
1: DNA is the genetic material • Early in the 20 th century, the identification of the molecules of inheritance loomed as a major challenge to biologists
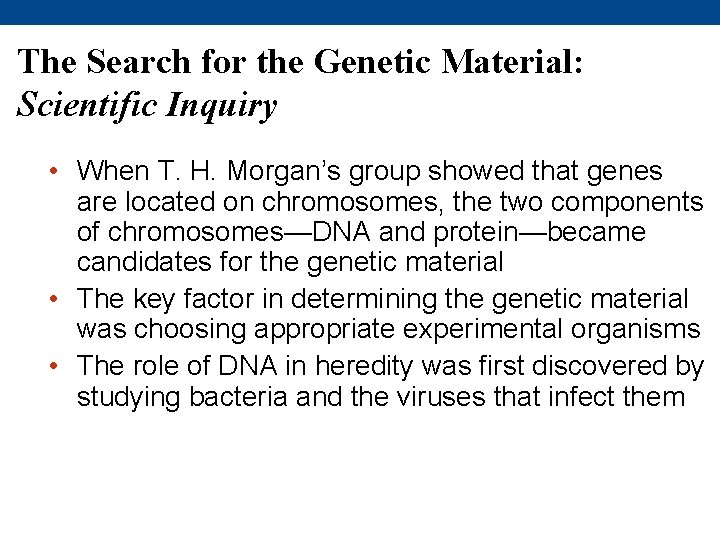
The Search for the Genetic Material: Scientific Inquiry • When T. H. Morgan’s group showed that genes are located on chromosomes, the two components of chromosomes—DNA and protein—became candidates for the genetic material • The key factor in determining the genetic material was choosing appropriate experimental organisms • The role of DNA in heredity was first discovered by studying bacteria and the viruses that infect them
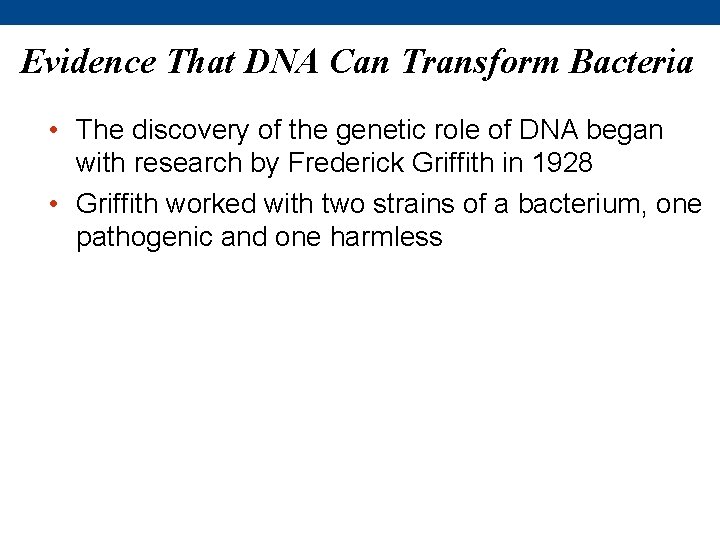
Evidence That DNA Can Transform Bacteria • The discovery of the genetic role of DNA began with research by Frederick Griffith in 1928 • Griffith worked with two strains of a bacterium, one pathogenic and one harmless
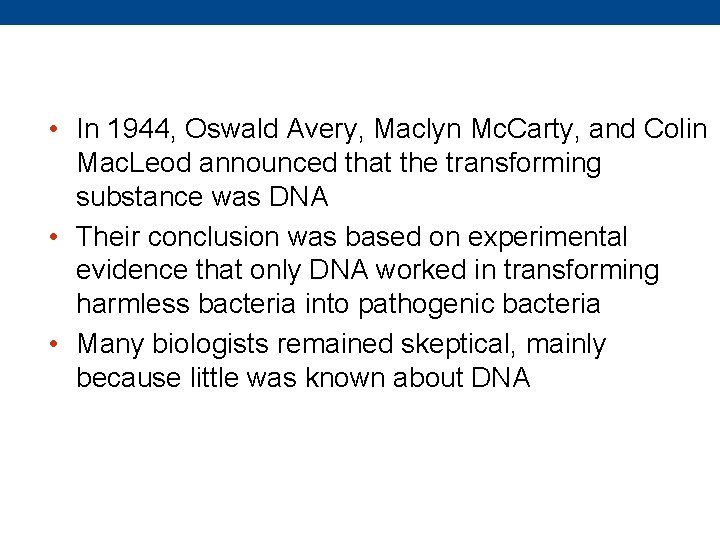
• In 1944, Oswald Avery, Maclyn Mc. Carty, and Colin Mac. Leod announced that the transforming substance was DNA • Their conclusion was based on experimental evidence that only DNA worked in transforming harmless bacteria into pathogenic bacteria • Many biologists remained skeptical, mainly because little was known about DNA
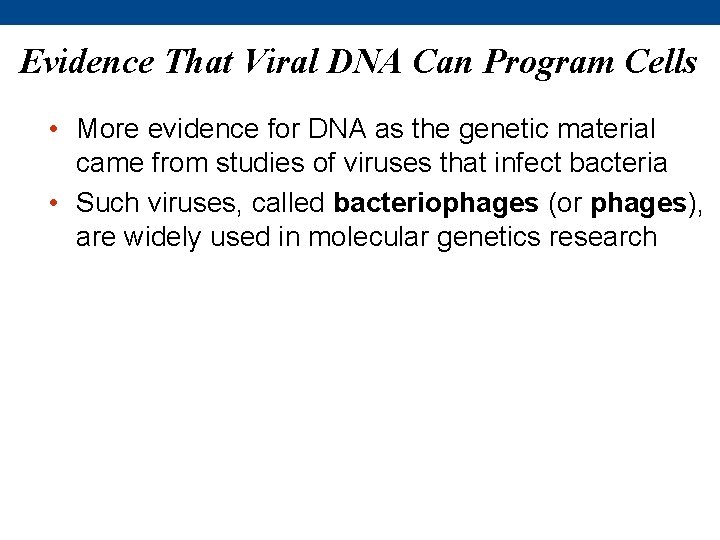
Evidence That Viral DNA Can Program Cells • More evidence for DNA as the genetic material came from studies of viruses that infect bacteria • Such viruses, called bacteriophages (or phages), are widely used in molecular genetics research
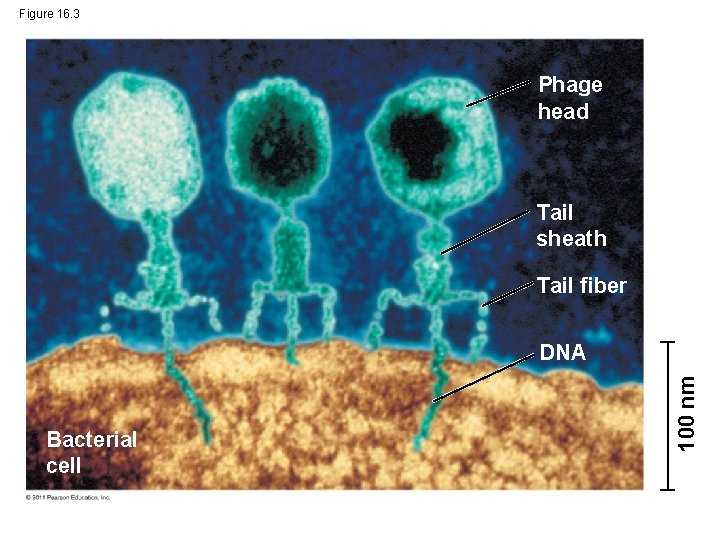
Figure 16. 3 Phage head Tail sheath Tail fiber Bacterial cell 100 nm DNA
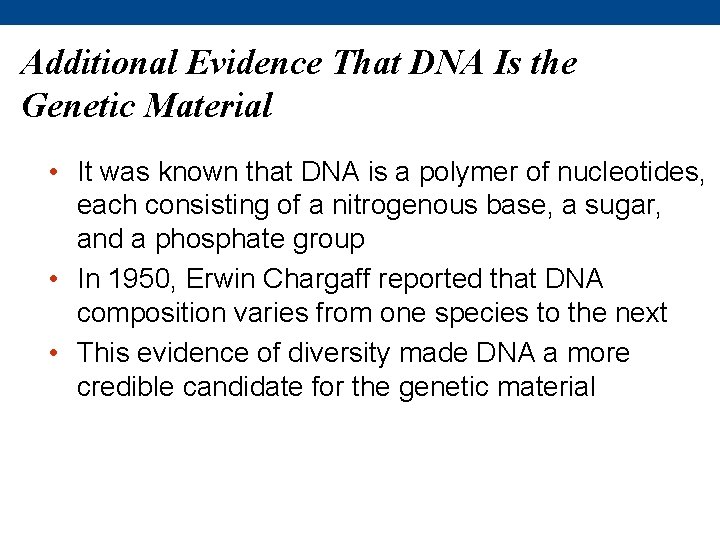
Additional Evidence That DNA Is the Genetic Material • It was known that DNA is a polymer of nucleotides, each consisting of a nitrogenous base, a sugar, and a phosphate group • In 1950, Erwin Chargaff reported that DNA composition varies from one species to the next • This evidence of diversity made DNA a more credible candidate for the genetic material
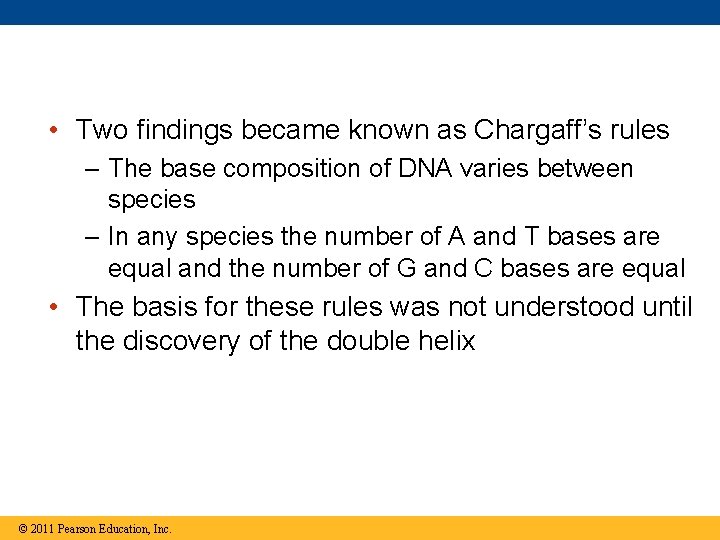
• Two findings became known as Chargaff’s rules – The base composition of DNA varies between species – In any species the number of A and T bases are equal and the number of G and C bases are equal • The basis for these rules was not understood until the discovery of the double helix © 2011 Pearson Education, Inc.
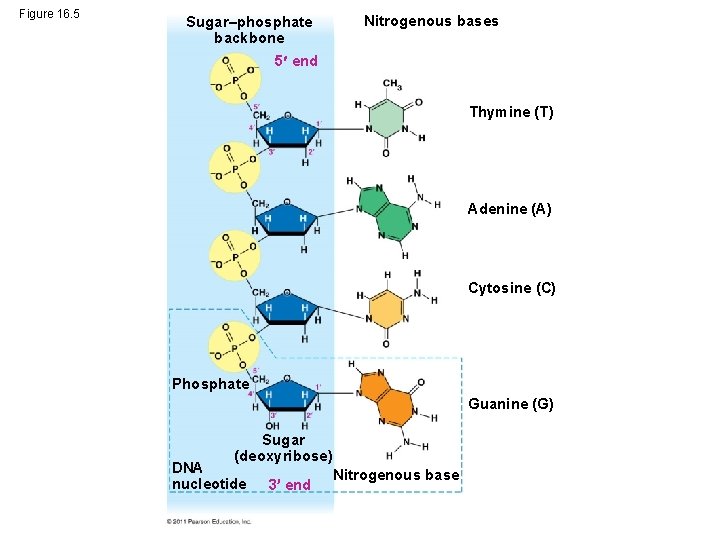
Figure 16. 5 Sugar–phosphate backbone Nitrogenous bases 5 end Thymine (T) Adenine (A) Cytosine (C) Phosphate Guanine (G) Sugar (deoxyribose) DNA nucleotide 3 end Nitrogenous base
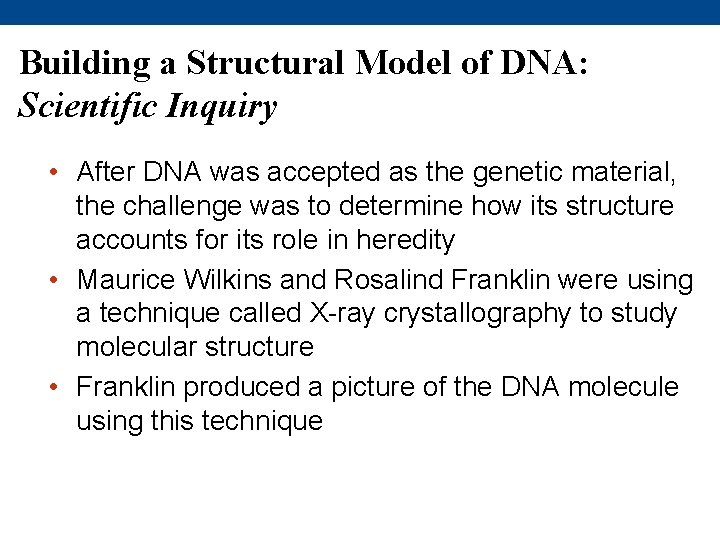
Building a Structural Model of DNA: Scientific Inquiry • After DNA was accepted as the genetic material, the challenge was to determine how its structure accounts for its role in heredity • Maurice Wilkins and Rosalind Franklin were using a technique called X-ray crystallography to study molecular structure • Franklin produced a picture of the DNA molecule using this technique
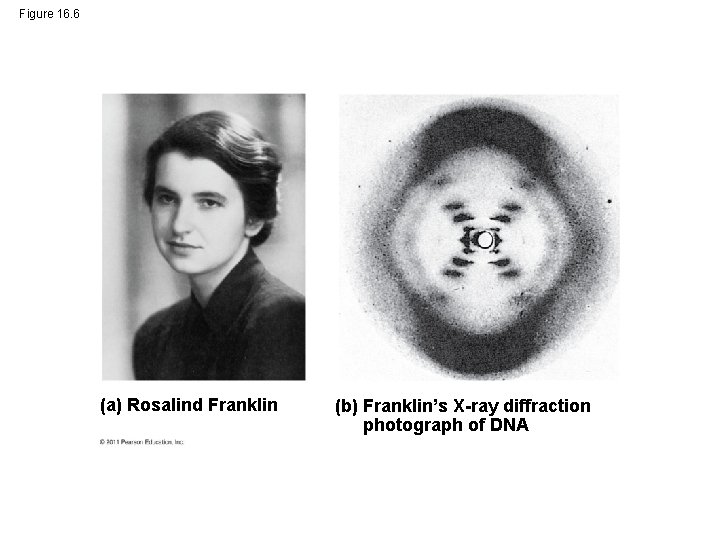
Figure 16. 6 (a) Rosalind Franklin (b) Franklin’s X-ray diffraction photograph of DNA
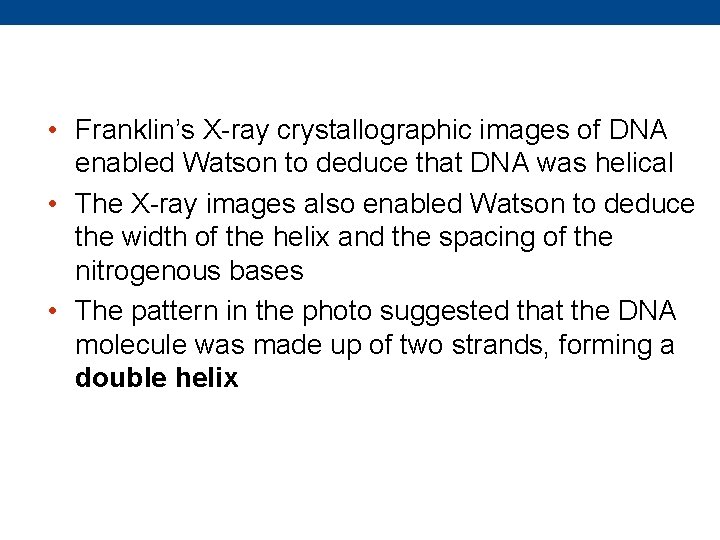
• Franklin’s X-ray crystallographic images of DNA enabled Watson to deduce that DNA was helical • The X-ray images also enabled Watson to deduce the width of the helix and the spacing of the nitrogenous bases • The pattern in the photo suggested that the DNA molecule was made up of two strands, forming a double helix
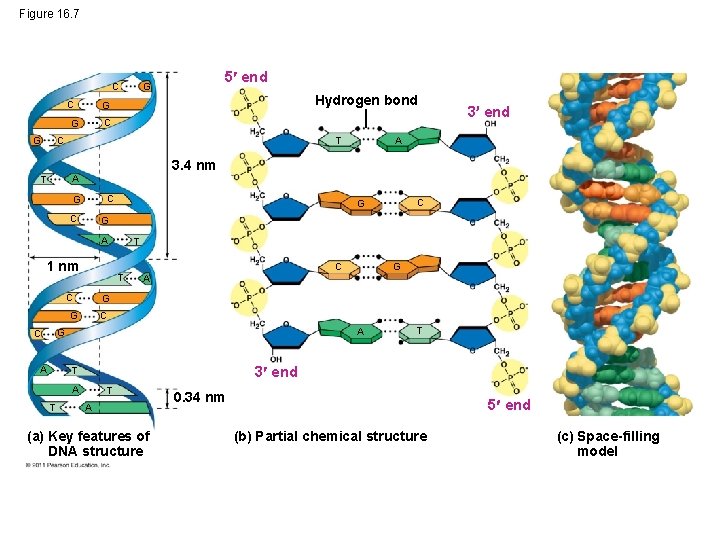
Figure 16. 7 C 5 end G C Hydrogen bond G C G 3. 4 nm C G 1 nm T T C C A G T 3 end T A T G C A G G A C A T 3 end T A (a) Key features of DNA structure 0. 34 nm 5 end (b) Partial chemical structure (c) Space-filling model
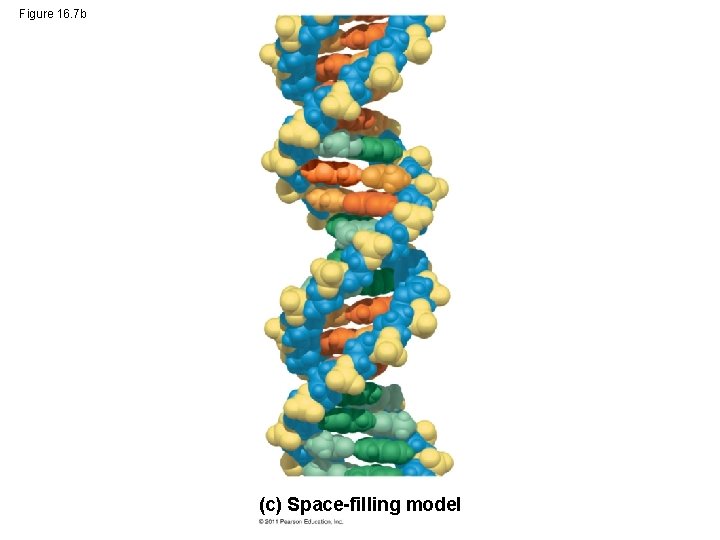
Figure 16. 7 b (c) Space-filling model
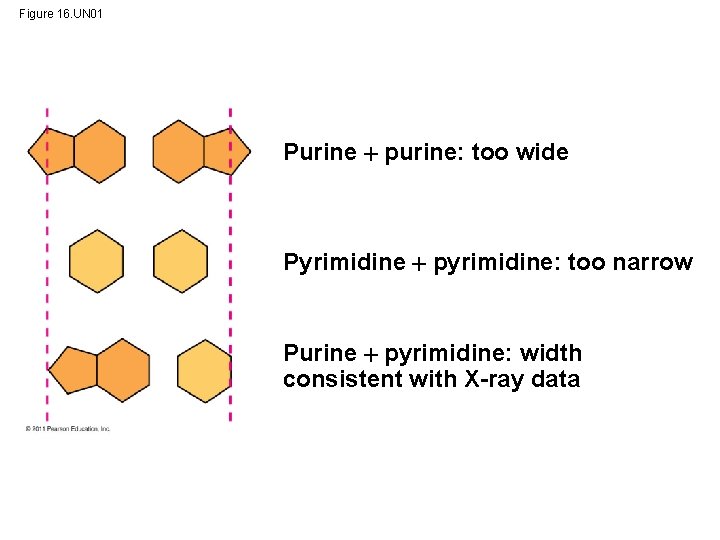
Figure 16. UN 01 Purine purine: too wide Pyrimidine pyrimidine: too narrow Purine pyrimidine: width consistent with X-ray data
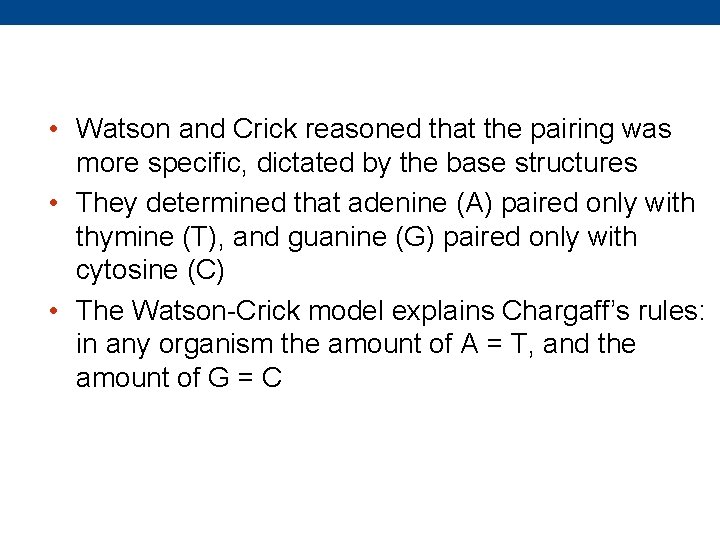
• Watson and Crick reasoned that the pairing was more specific, dictated by the base structures • They determined that adenine (A) paired only with thymine (T), and guanine (G) paired only with cytosine (C) • The Watson-Crick model explains Chargaff’s rules: in any organism the amount of A = T, and the amount of G = C
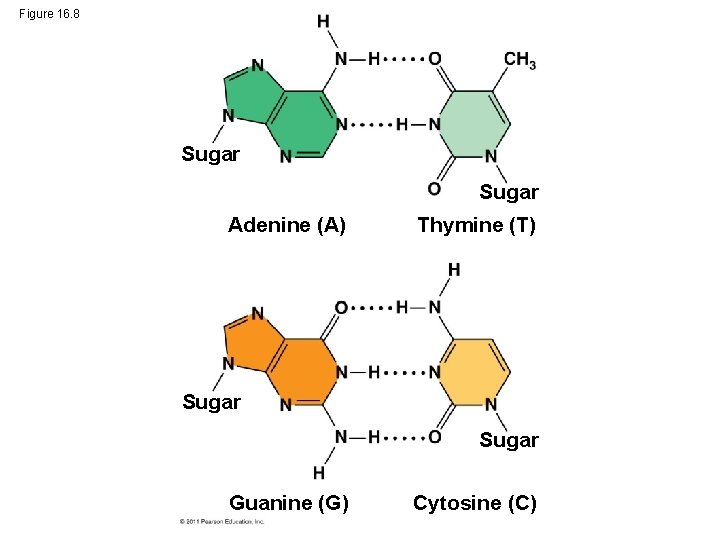
Figure 16. 8 Sugar Adenine (A) Thymine (T) Sugar Guanine (G) Cytosine (C)
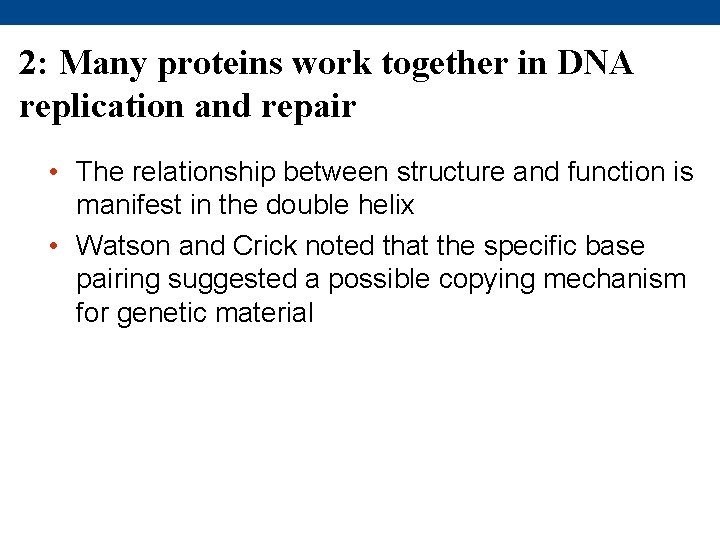
2: Many proteins work together in DNA replication and repair • The relationship between structure and function is manifest in the double helix • Watson and Crick noted that the specific base pairing suggested a possible copying mechanism for genetic material
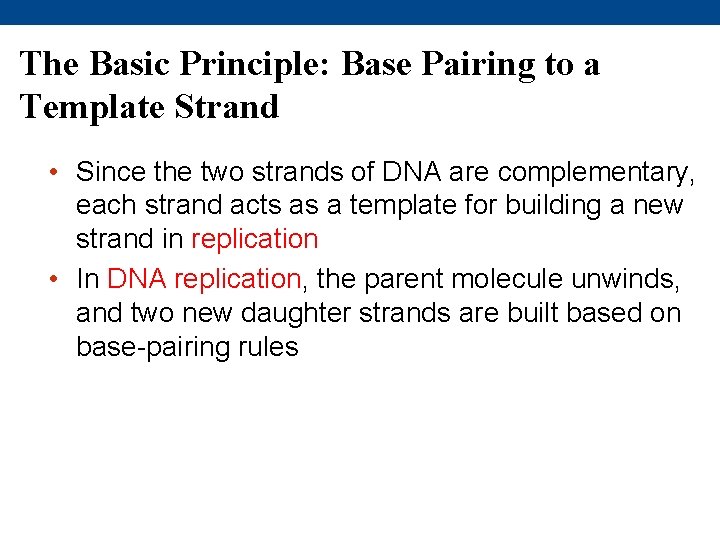
The Basic Principle: Base Pairing to a Template Strand • Since the two strands of DNA are complementary, each strand acts as a template for building a new strand in replication • In DNA replication, the parent molecule unwinds, and two new daughter strands are built based on base-pairing rules
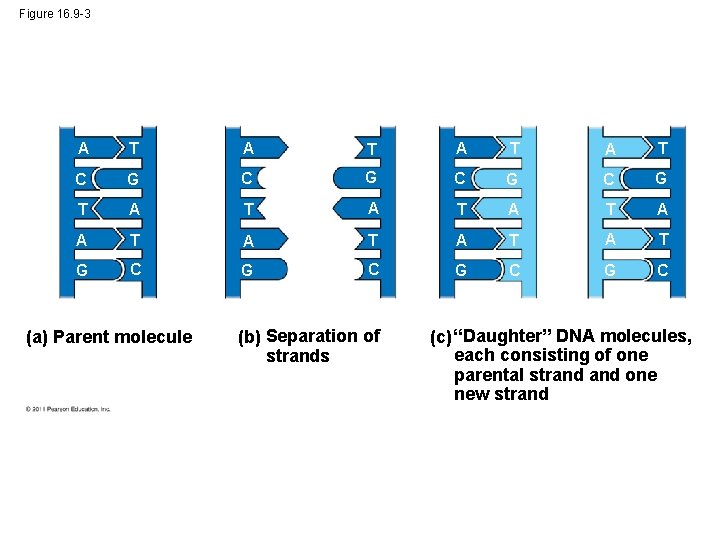
Figure 16. 9 -3 A T A T C G C G T A T A T G C G C (a) Parent molecule (b) Separation of strands (c) “Daughter” DNA molecules, each consisting of one parental strand one new strand
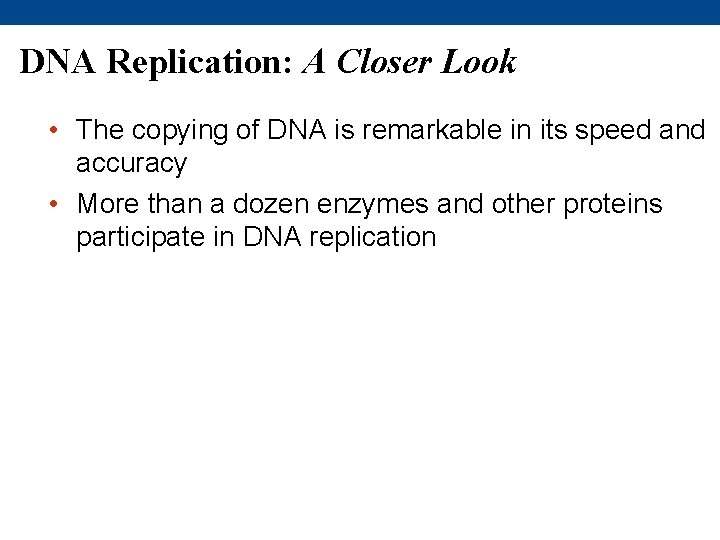
DNA Replication: A Closer Look • The copying of DNA is remarkable in its speed and accuracy • More than a dozen enzymes and other proteins participate in DNA replication
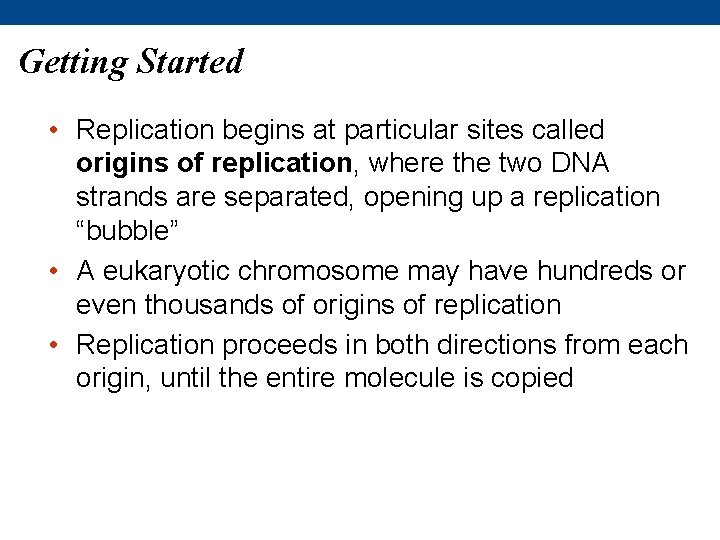
Getting Started • Replication begins at particular sites called origins of replication, where the two DNA strands are separated, opening up a replication “bubble” • A eukaryotic chromosome may have hundreds or even thousands of origins of replication • Replication proceeds in both directions from each origin, until the entire molecule is copied
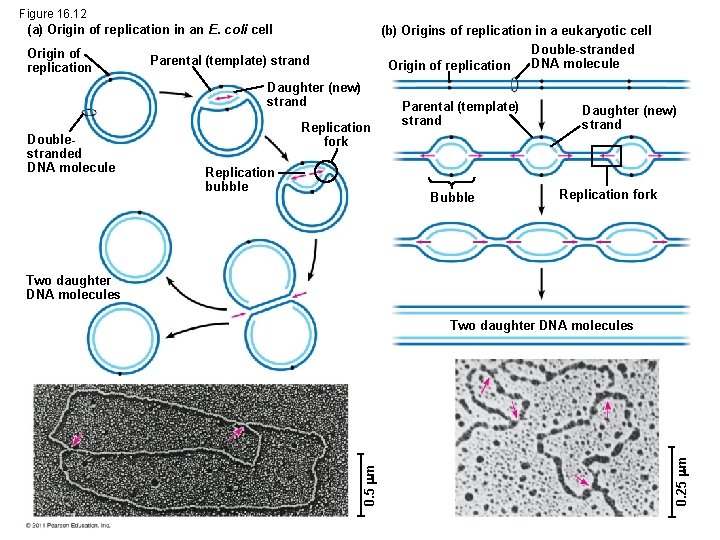
Figure 16. 12 (a) Origin of replication in an E. coli cell Origin of replication (b) Origins of replication in a eukaryotic cell Double-stranded DNA molecule Origin of replication Parental (template) strand Daughter (new) strand Doublestranded DNA molecule Replication fork Replication bubble Parental (template) strand Bubble Daughter (new) strand Replication fork Two daughter DNA molecules 0. 25 m 0. 5 m Two daughter DNA molecules
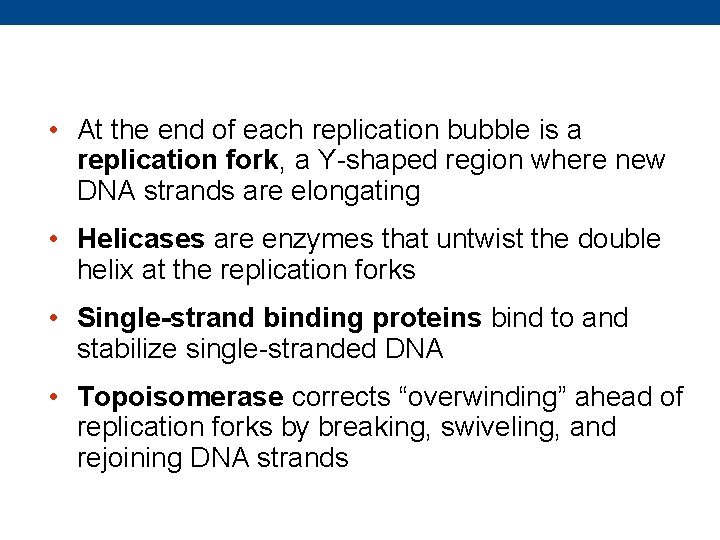
• At the end of each replication bubble is a replication fork, a Y-shaped region where new DNA strands are elongating • Helicases are enzymes that untwist the double helix at the replication forks • Single-strand binding proteins bind to and stabilize single-stranded DNA • Topoisomerase corrects “overwinding” ahead of replication forks by breaking, swiveling, and rejoining DNA strands
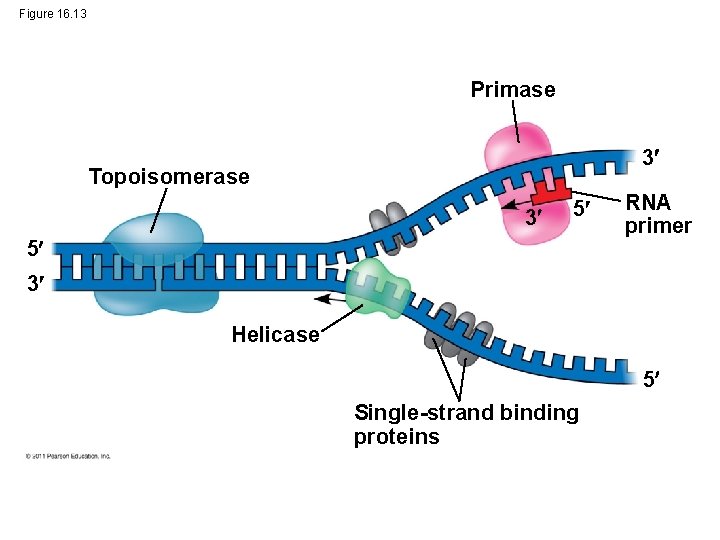
Figure 16. 13 Primase 3 Topoisomerase 3 5 5 RNA primer 3 Helicase 5 Single-strand binding proteins
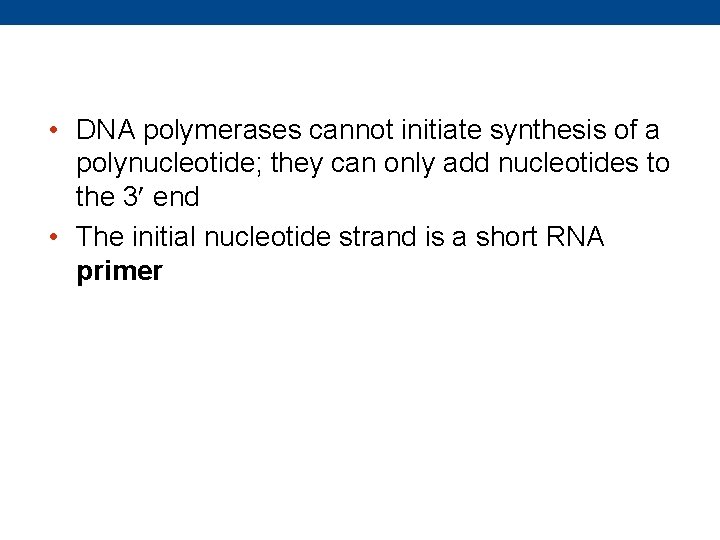
• DNA polymerases cannot initiate synthesis of a polynucleotide; they can only add nucleotides to the 3 end • The initial nucleotide strand is a short RNA primer
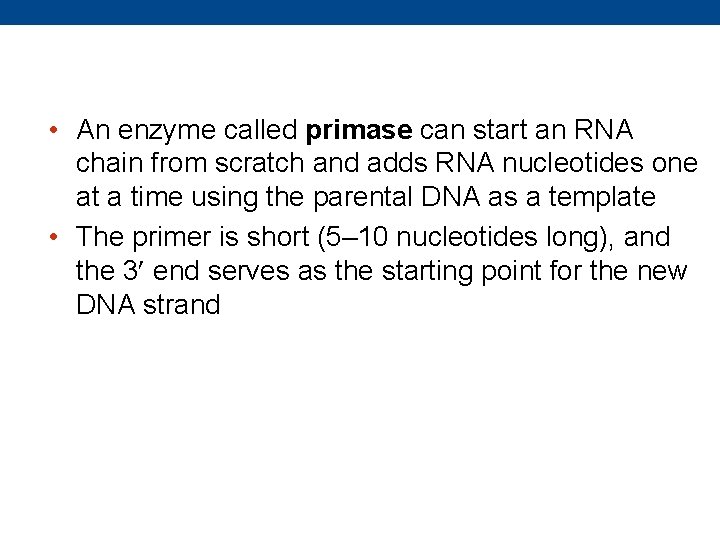
• An enzyme called primase can start an RNA chain from scratch and adds RNA nucleotides one at a time using the parental DNA as a template • The primer is short (5– 10 nucleotides long), and the 3 end serves as the starting point for the new DNA strand
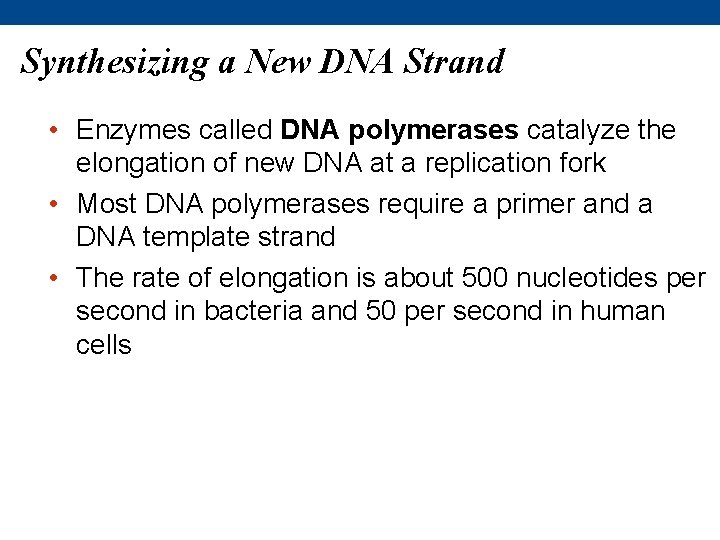
Synthesizing a New DNA Strand • Enzymes called DNA polymerases catalyze the elongation of new DNA at a replication fork • Most DNA polymerases require a primer and a DNA template strand • The rate of elongation is about 500 nucleotides per second in bacteria and 50 per second in human cells
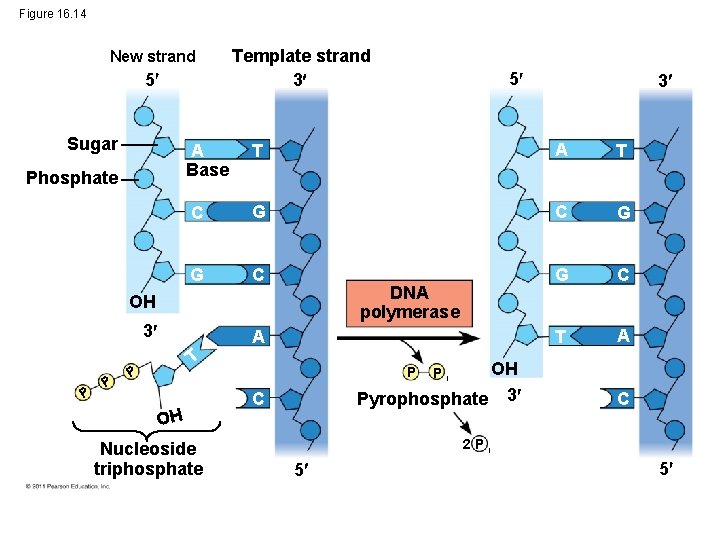
Figure 16. 14 New strand 5 Sugar Phosphate Template strand 3 T A T C G G C T A 3 P P 3 A Base DNA polymerase OH A T P 5 OH Nucleoside triphosphate OH Pyrophosphate 3 P C Pi C 2 Pi 5 5
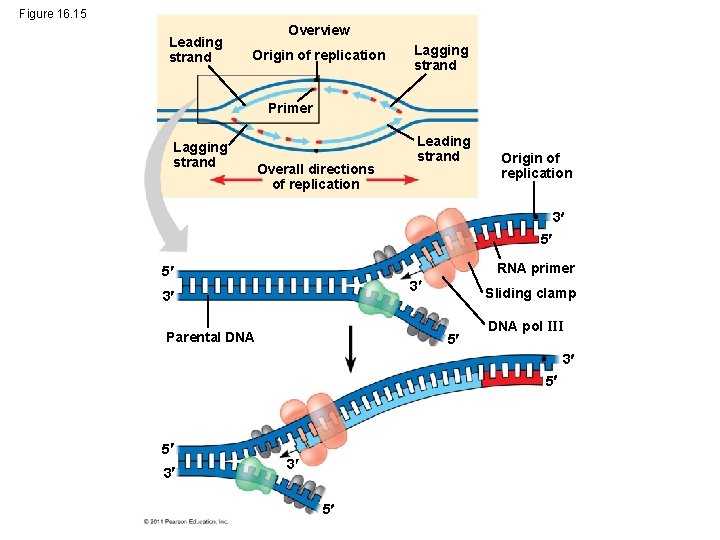
Figure 16. 15 Leading strand Overview Origin of replication Lagging strand Primer Lagging strand Overall directions of replication Leading strand Origin of replication 3 5 RNA primer 5 3 3 Parental DNA Sliding clamp 5 DNA pol III 3 5 5 3 3 5
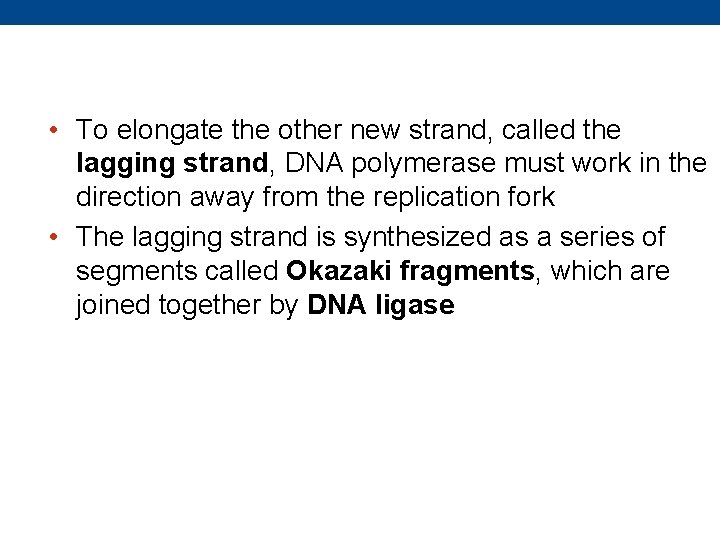
• To elongate the other new strand, called the lagging strand, DNA polymerase must work in the direction away from the replication fork • The lagging strand is synthesized as a series of segments called Okazaki fragments, which are joined together by DNA ligase
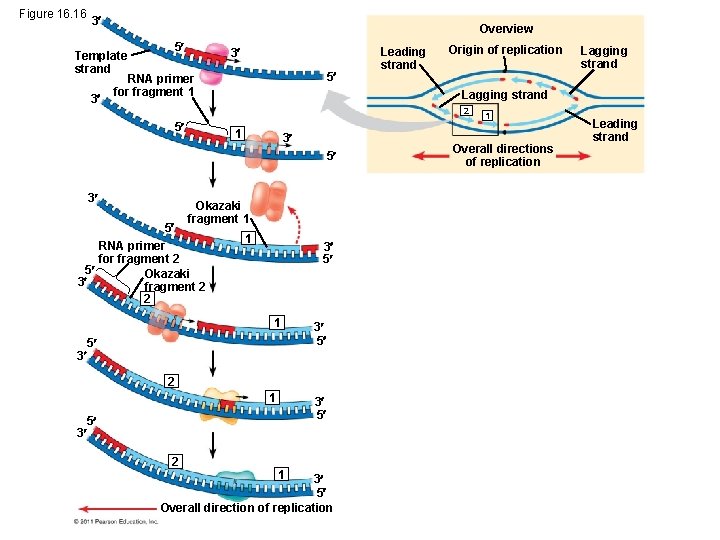
Figure 16. 16 3 Overview 5 Template strand 3 3 5 RNA primer for fragment 1 Leading strand Origin of replication Lagging strand 2 5 1 3 5 Okazaki fragment 1 RNA primer for fragment 2 5 Okazaki 3 fragment 2 2 1 3 5 1 3 5 3 5 2 1 3 3 5 5 2 Lagging strand 1 3 5 Overall direction of replication 1 Overall directions of replication Leading strand
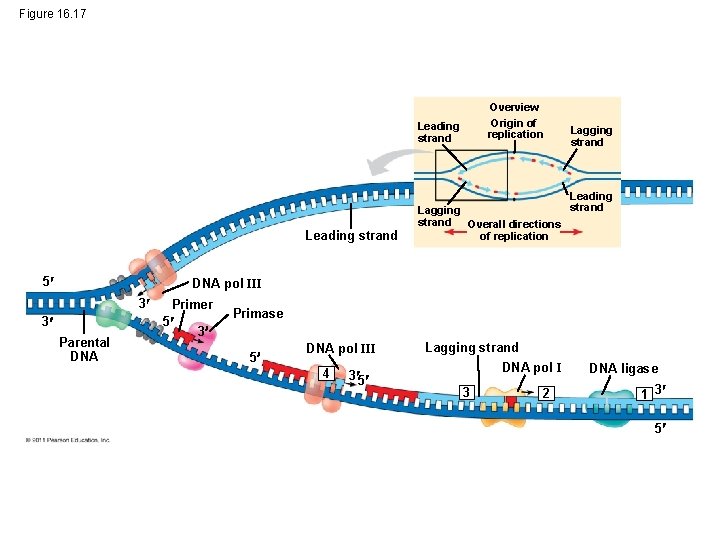
Figure 16. 17 Overview Origin of replication Leading strand 5 Lagging strand Overall directions of replication Lagging strand Leading strand DNA pol III 3 3 Parental DNA Primer 5 3 Primase 5 DNA pol III 4 3 5 Lagging strand DNA pol I 3 2 DNA ligase 1 3 5
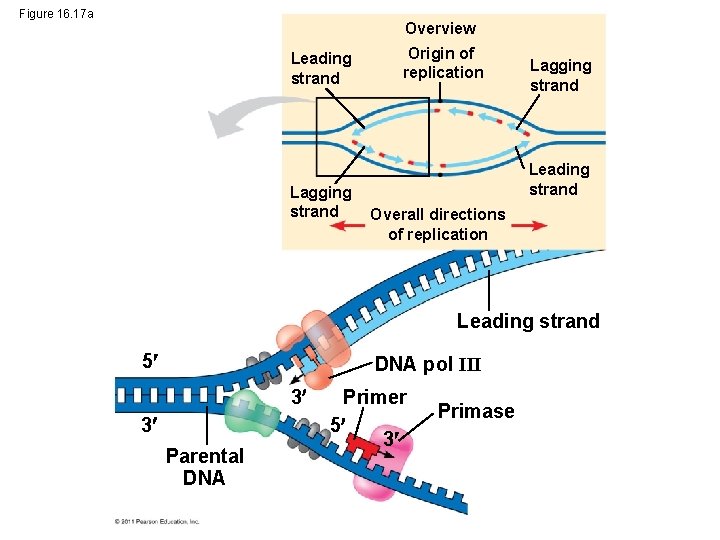
Figure 16. 17 a Overview Leading strand Lagging strand Origin of replication Lagging strand Leading strand Overall directions of replication Leading strand 5 DNA pol III 3 3 Parental DNA Primer 5 3 Primase
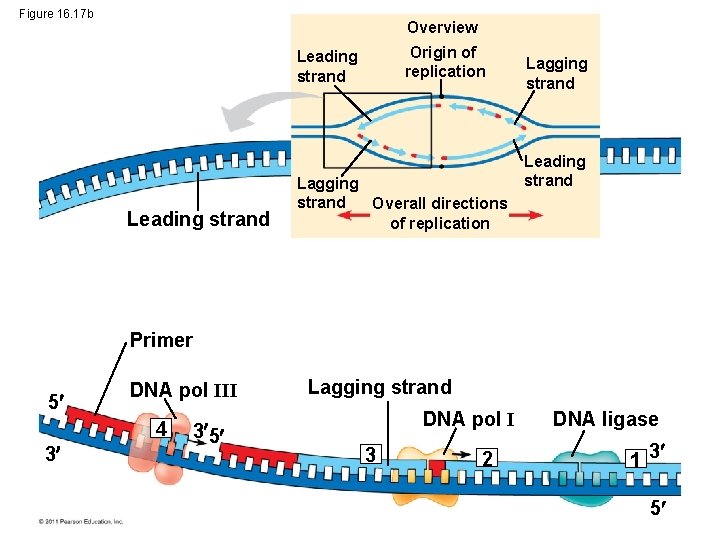
Figure 16. 17 b Overview Origin of replication Leading strand Lagging strand Overall directions of replication Lagging strand Leading strand Primer 5 DNA pol III 4 3 3 5 Lagging strand DNA pol I 3 2 DNA ligase 1 3 5
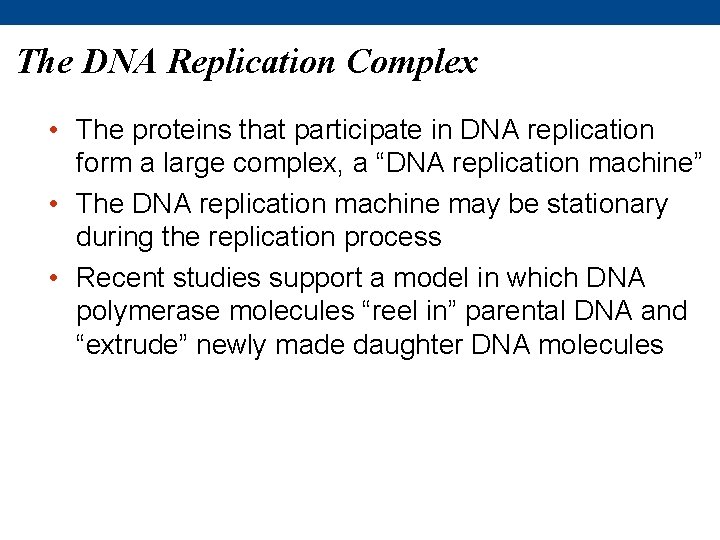
The DNA Replication Complex • The proteins that participate in DNA replication form a large complex, a “DNA replication machine” • The DNA replication machine may be stationary during the replication process • Recent studies support a model in which DNA polymerase molecules “reel in” parental DNA and “extrude” newly made daughter DNA molecules
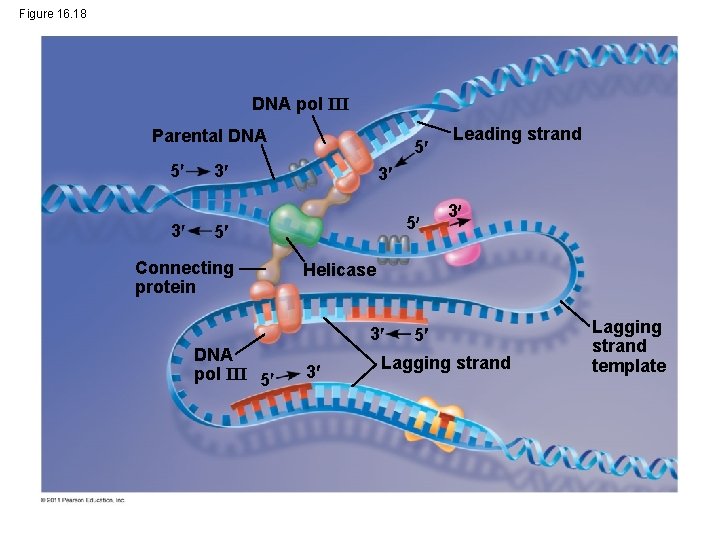
Figure 16. 18 DNA pol III Parental DNA 5 3 5 3 3 5 5 Connecting protein 3 Helicase 3 DNA pol III 5 Leading strand 3 5 Lagging strand template
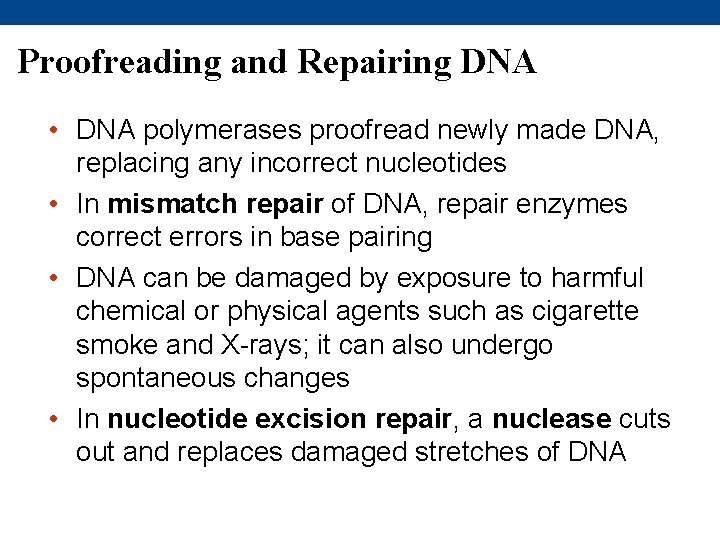
Proofreading and Repairing DNA • DNA polymerases proofread newly made DNA, replacing any incorrect nucleotides • In mismatch repair of DNA, repair enzymes correct errors in base pairing • DNA can be damaged by exposure to harmful chemical or physical agents such as cigarette smoke and X-rays; it can also undergo spontaneous changes • In nucleotide excision repair, a nuclease cuts out and replaces damaged stretches of DNA
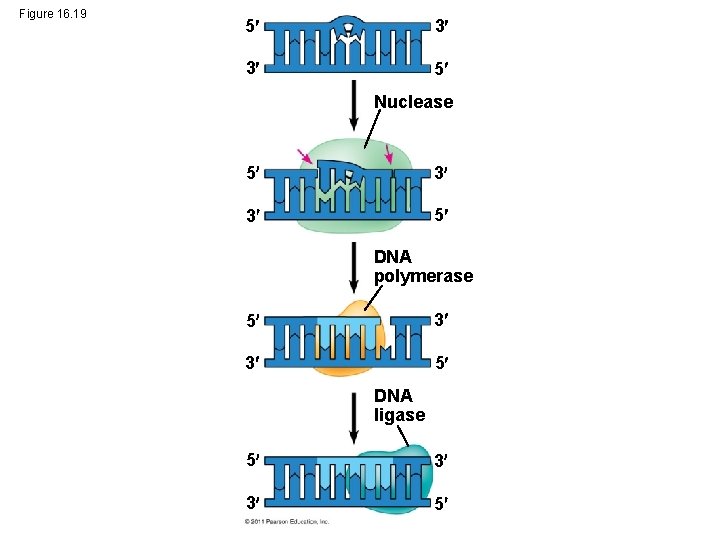
Figure 16. 19 5 3 3 5 Nuclease 5 3 3 5 DNA polymerase 5 3 3 5 DNA ligase 5 3 3 5
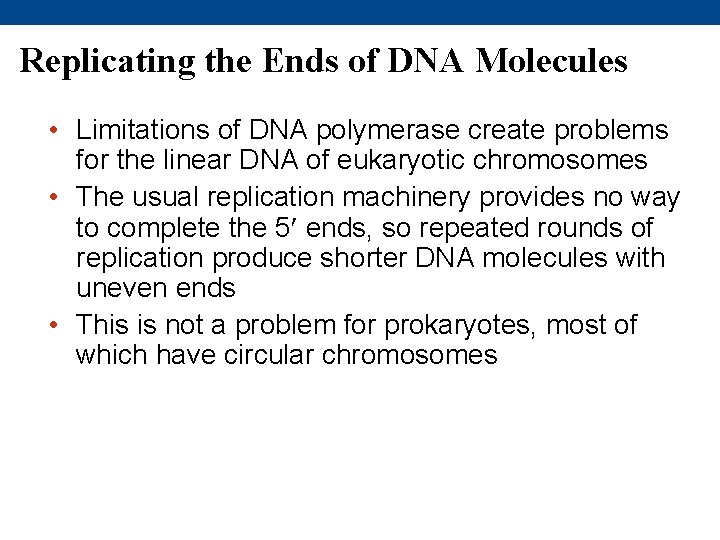
Replicating the Ends of DNA Molecules • Limitations of DNA polymerase create problems for the linear DNA of eukaryotic chromosomes • The usual replication machinery provides no way to complete the 5 ends, so repeated rounds of replication produce shorter DNA molecules with uneven ends • This is not a problem for prokaryotes, most of which have circular chromosomes
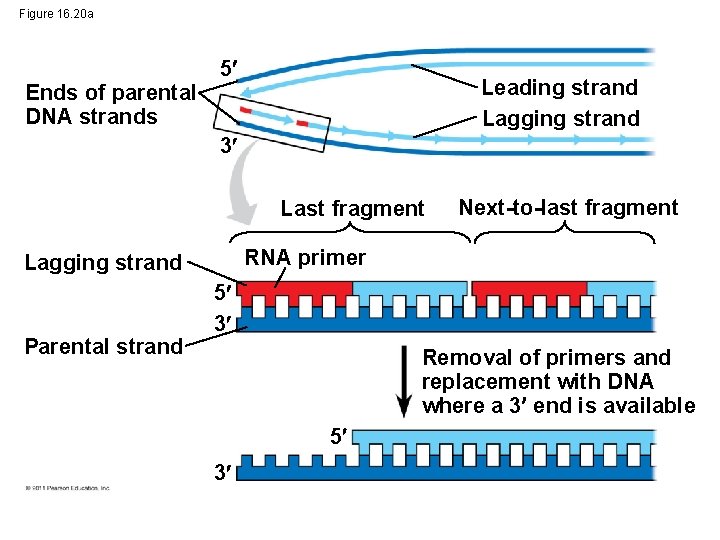
Figure 16. 20 a Ends of parental DNA strands 5 Leading strand Lagging strand 3 Last fragment RNA primer Lagging strand Parental strand Next-to-last fragment 5 3 Removal of primers and replacement with DNA where a 3 end is available 5 3
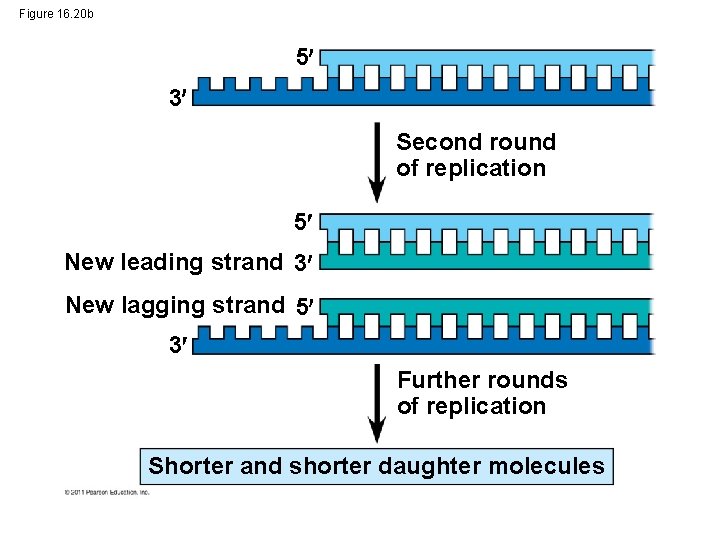
Figure 16. 20 b 5 3 Second round of replication 5 New leading strand 3 New lagging strand 5 3 Further rounds of replication Shorter and shorter daughter molecules
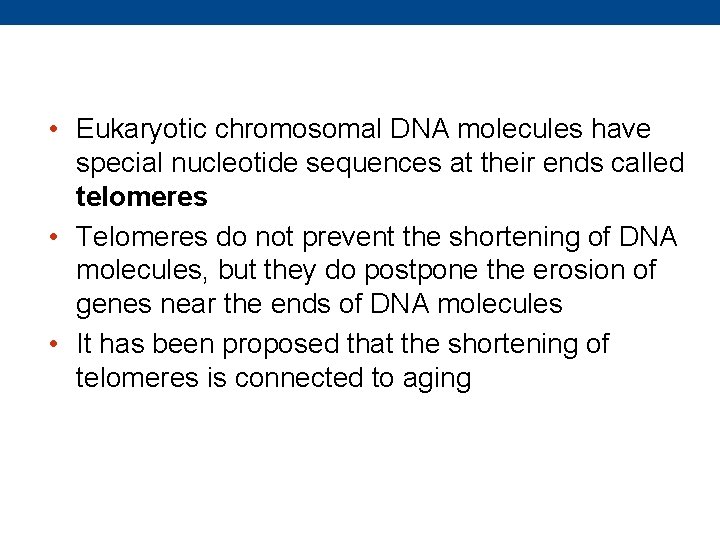
• Eukaryotic chromosomal DNA molecules have special nucleotide sequences at their ends called telomeres • Telomeres do not prevent the shortening of DNA molecules, but they do postpone the erosion of genes near the ends of DNA molecules • It has been proposed that the shortening of telomeres is connected to aging
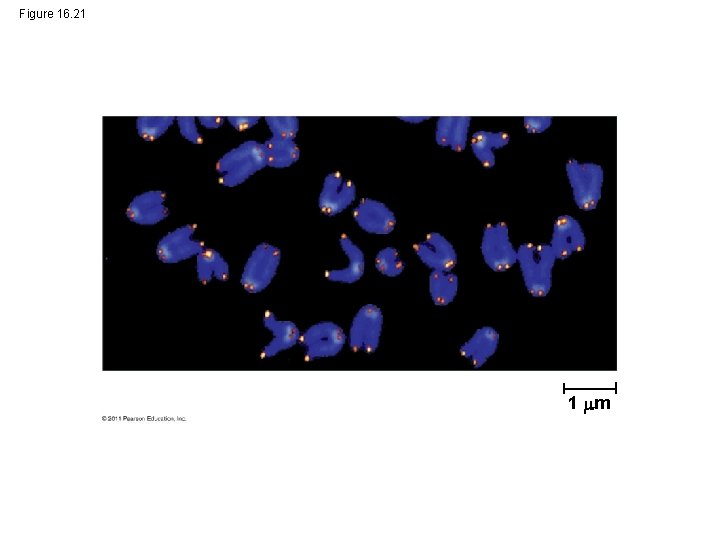
Figure 16. 21 1 m
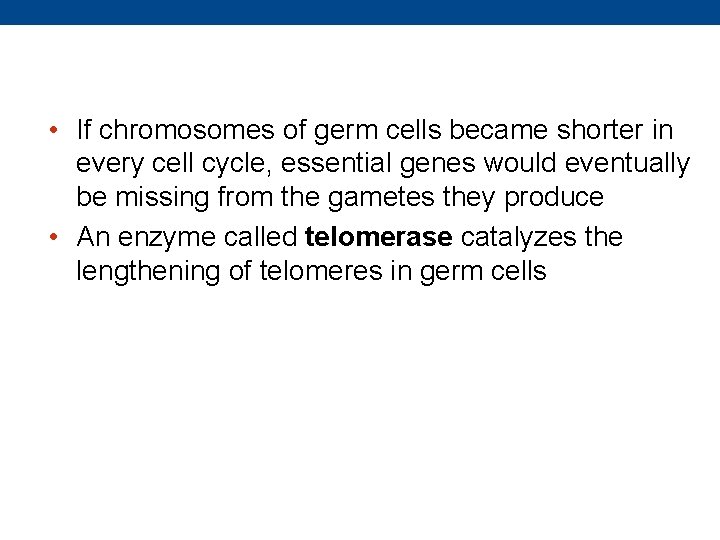
• If chromosomes of germ cells became shorter in every cell cycle, essential genes would eventually be missing from the gametes they produce • An enzyme called telomerase catalyzes the lengthening of telomeres in germ cells
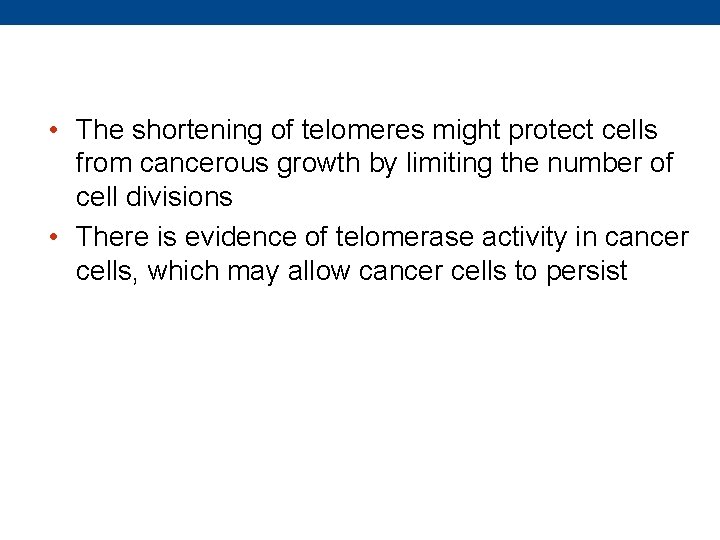
• The shortening of telomeres might protect cells from cancerous growth by limiting the number of cell divisions • There is evidence of telomerase activity in cancer cells, which may allow cancer cells to persist
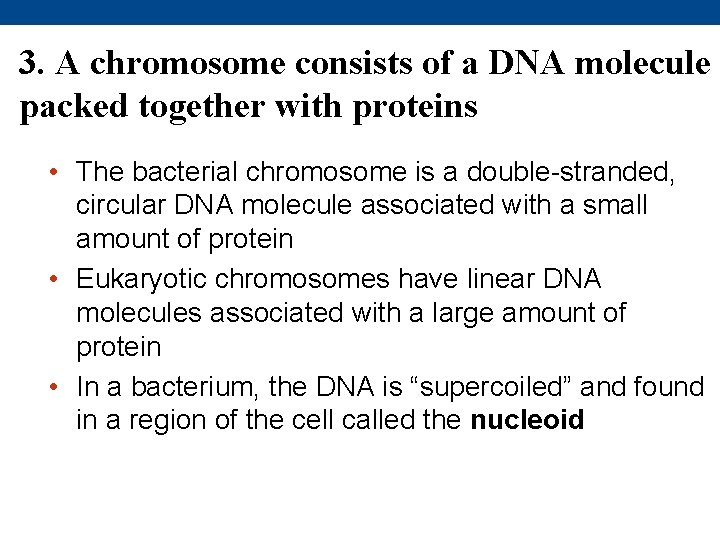
3. A chromosome consists of a DNA molecule packed together with proteins • The bacterial chromosome is a double-stranded, circular DNA molecule associated with a small amount of protein • Eukaryotic chromosomes have linear DNA molecules associated with a large amount of protein • In a bacterium, the DNA is “supercoiled” and found in a region of the cell called the nucleoid
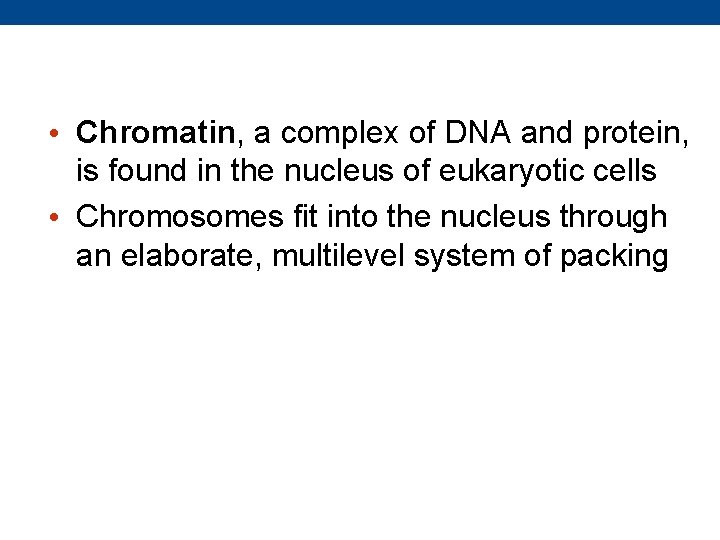
• Chromatin, a complex of DNA and protein, is found in the nucleus of eukaryotic cells • Chromosomes fit into the nucleus through an elaborate, multilevel system of packing
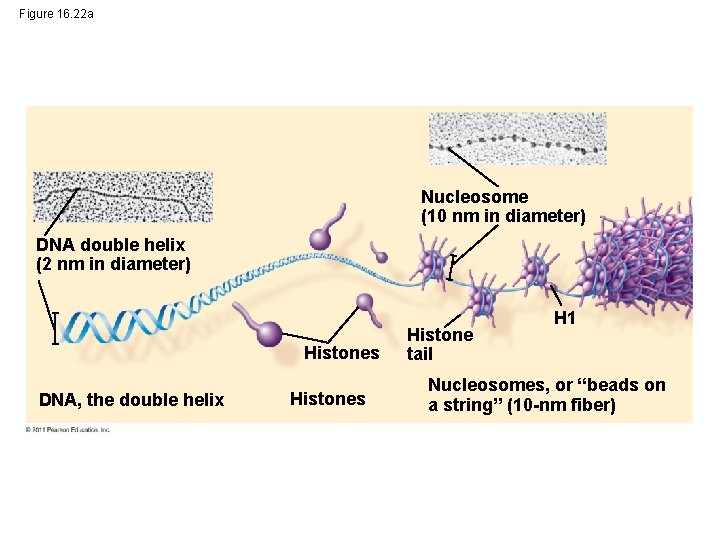
Figure 16. 22 a Nucleosome (10 nm in diameter) DNA double helix (2 nm in diameter) Histones DNA, the double helix Histones Histone tail H 1 Nucleosomes, or “beads on a string” (10 -nm fiber)
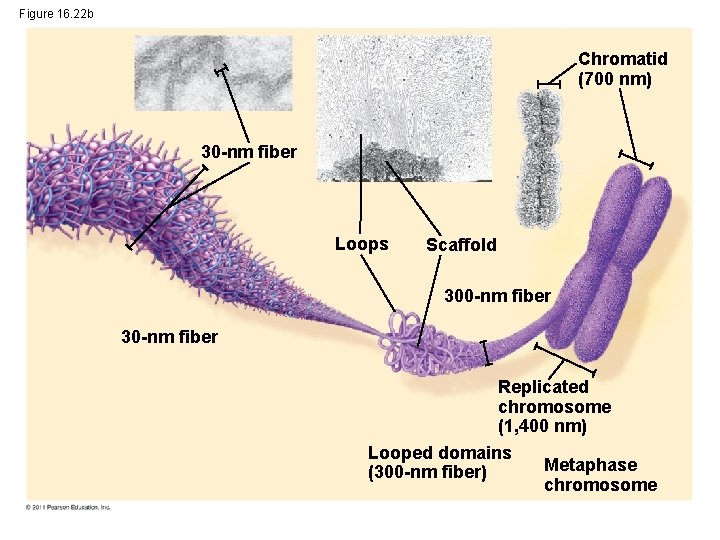
Figure 16. 22 b Chromatid (700 nm) 30 -nm fiber Loops Scaffold 300 -nm fiber 30 -nm fiber Replicated chromosome (1, 400 nm) Looped domains Metaphase (300 -nm fiber) chromosome
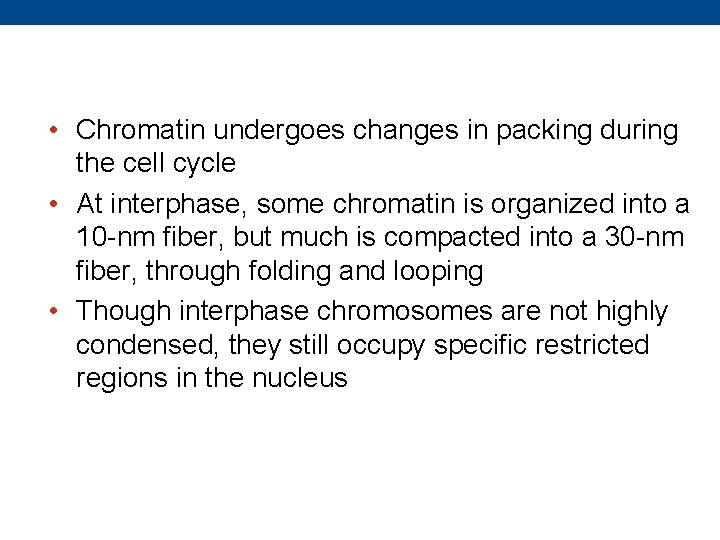
• Chromatin undergoes changes in packing during the cell cycle • At interphase, some chromatin is organized into a 10 -nm fiber, but much is compacted into a 30 -nm fiber, through folding and looping • Though interphase chromosomes are not highly condensed, they still occupy specific restricted regions in the nucleus
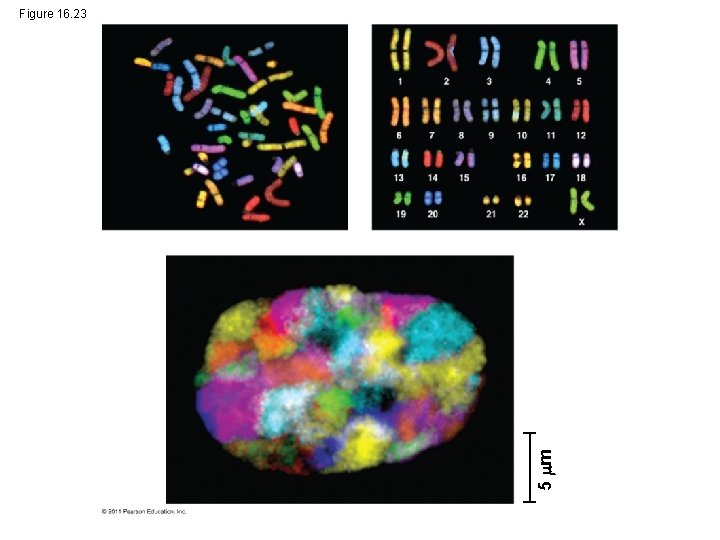
5 m Figure 16. 23
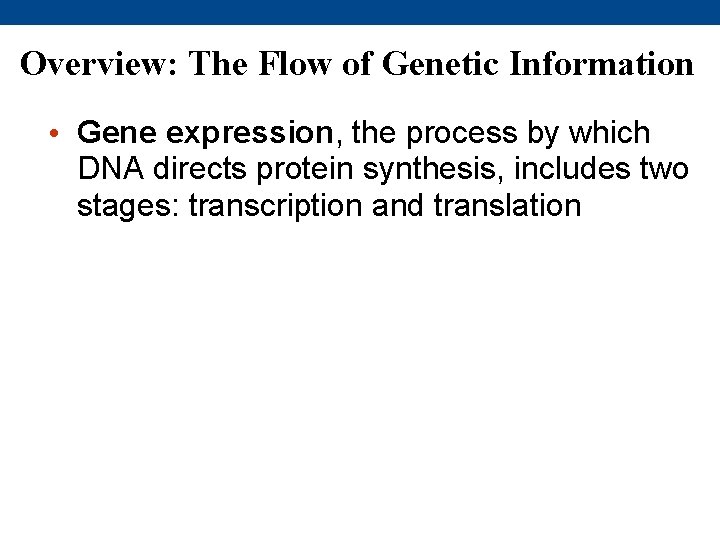
Overview: The Flow of Genetic Information • Gene expression, the process by which DNA directs protein synthesis, includes two stages: transcription and translation
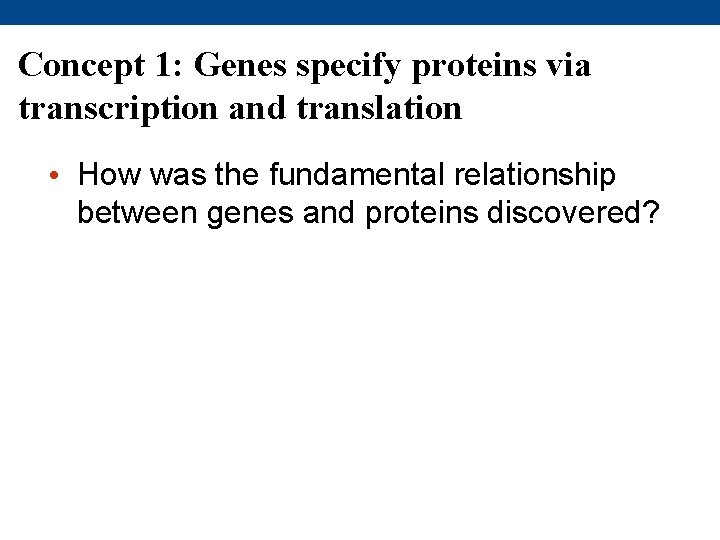
Concept 1: Genes specify proteins via transcription and translation • How was the fundamental relationship between genes and proteins discovered?
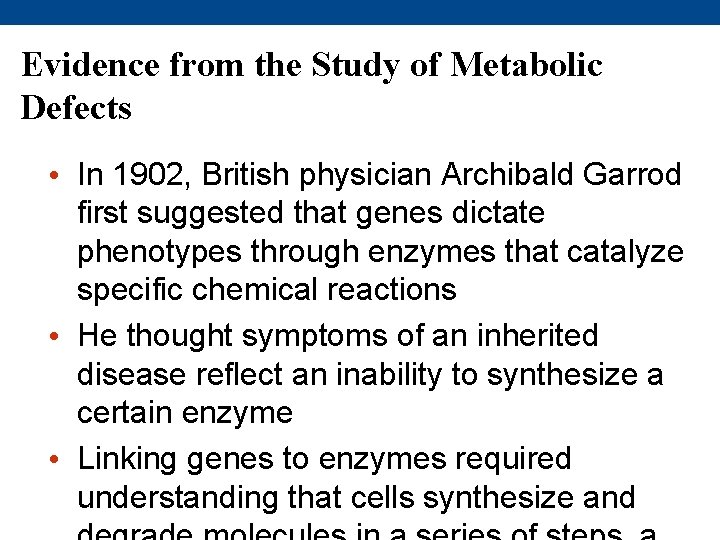
Evidence from the Study of Metabolic Defects • In 1902, British physician Archibald Garrod first suggested that genes dictate phenotypes through enzymes that catalyze specific chemical reactions • He thought symptoms of an inherited disease reflect an inability to synthesize a certain enzyme • Linking genes to enzymes required understanding that cells synthesize and
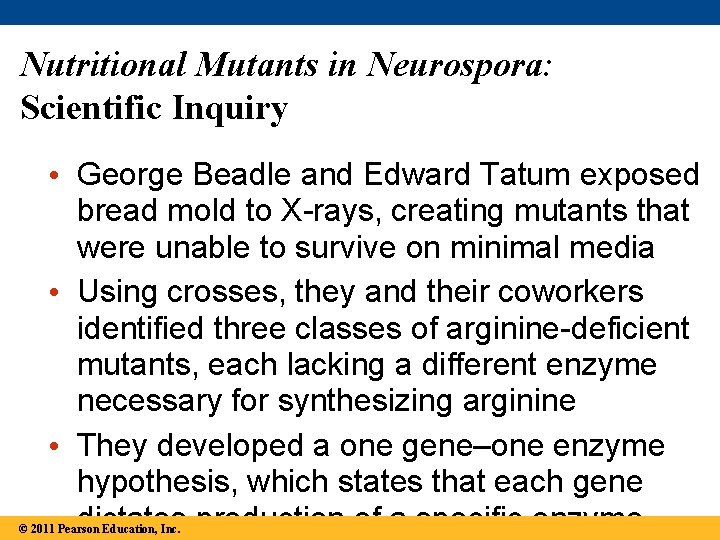
Nutritional Mutants in Neurospora: Scientific Inquiry • George Beadle and Edward Tatum exposed bread mold to X-rays, creating mutants that were unable to survive on minimal media • Using crosses, they and their coworkers identified three classes of arginine-deficient mutants, each lacking a different enzyme necessary for synthesizing arginine • They developed a one gene–one enzyme hypothesis, which states that each gene dictates production of a specific enzyme © 2011 Pearson Education, Inc.
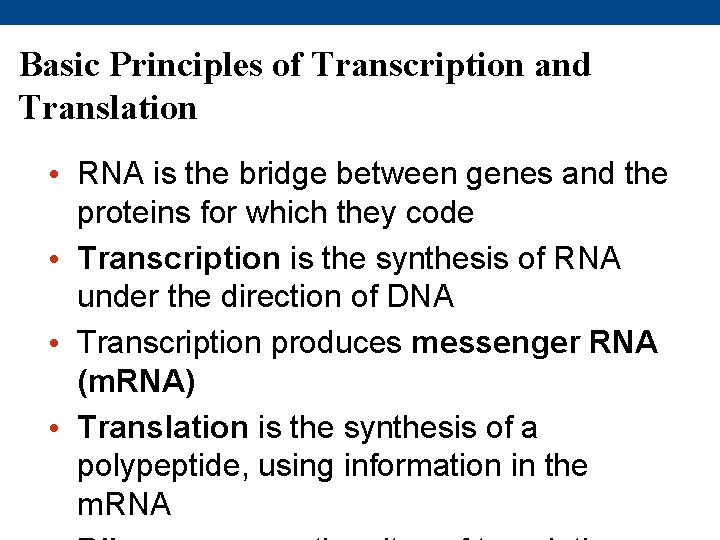
Basic Principles of Transcription and Translation • RNA is the bridge between genes and the proteins for which they code • Transcription is the synthesis of RNA under the direction of DNA • Transcription produces messenger RNA (m. RNA) • Translation is the synthesis of a polypeptide, using information in the m. RNA
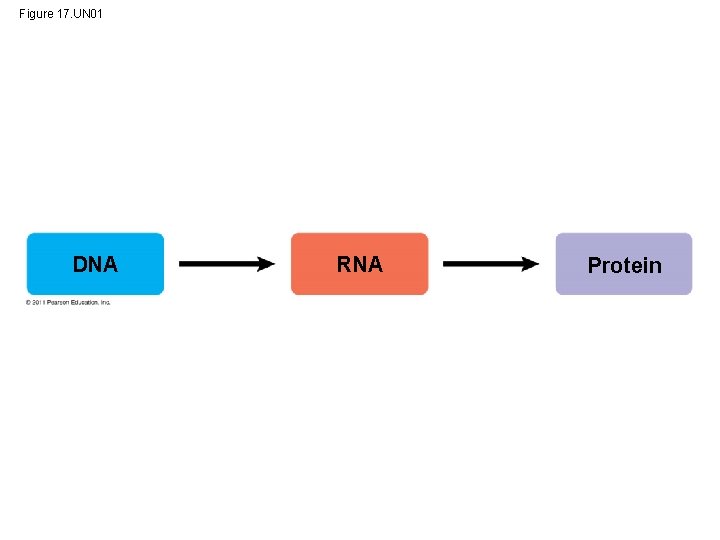
Figure 17. UN 01 DNA RNA Protein
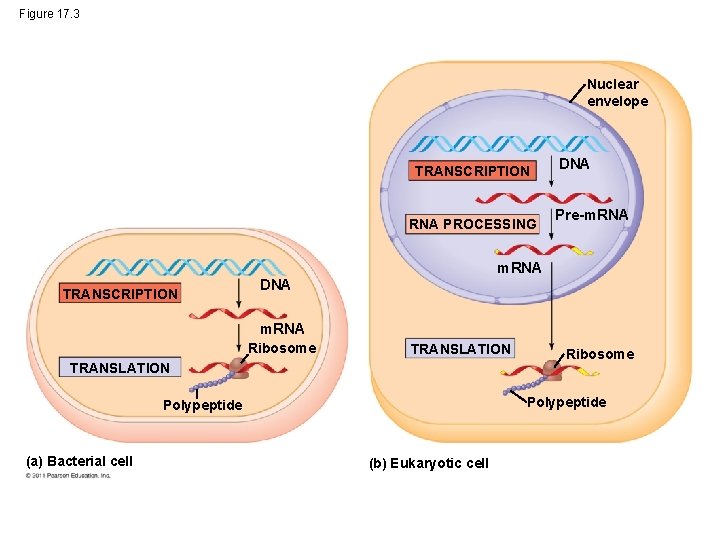
Figure 17. 3 Nuclear envelope TRANSCRIPTION RNA PROCESSING DNA Pre-m. RNA TRANSCRIPTION DNA m. RNA Ribosome TRANSLATION Polypeptide (a) Bacterial cell Ribosome (b) Eukaryotic cell
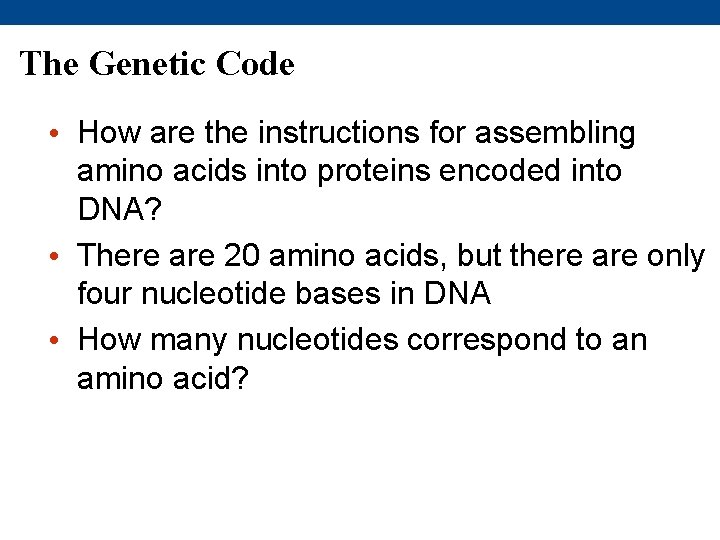
The Genetic Code • How are the instructions for assembling amino acids into proteins encoded into DNA? • There are 20 amino acids, but there are only four nucleotide bases in DNA • How many nucleotides correspond to an amino acid?
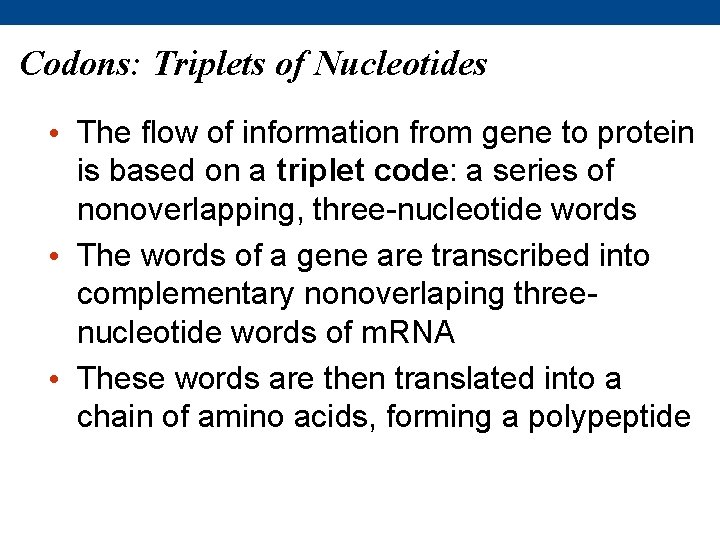
Codons: Triplets of Nucleotides • The flow of information from gene to protein is based on a triplet code: a series of nonoverlapping, three-nucleotide words • The words of a gene are transcribed into complementary nonoverlaping threenucleotide words of m. RNA • These words are then translated into a chain of amino acids, forming a polypeptide
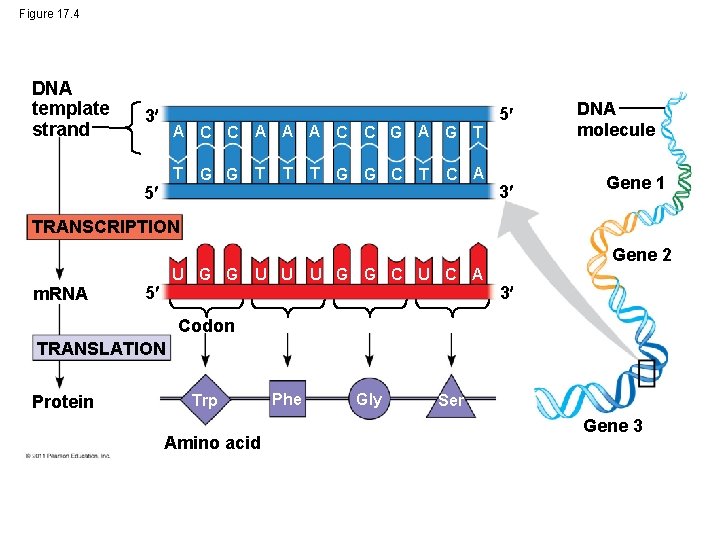
Figure 17. 4 DNA template strand 3 A C C A A A C T T 5 G G T T C G A G G G C T T C A 5 3 DNA molecule Gene 1 TRANSCRIPTION Gene 2 m. RNA U G G 5 U U U G G C U C A 3 Codon TRANSLATION Protein Trp Amino acid Phe Gly Ser Gene 3
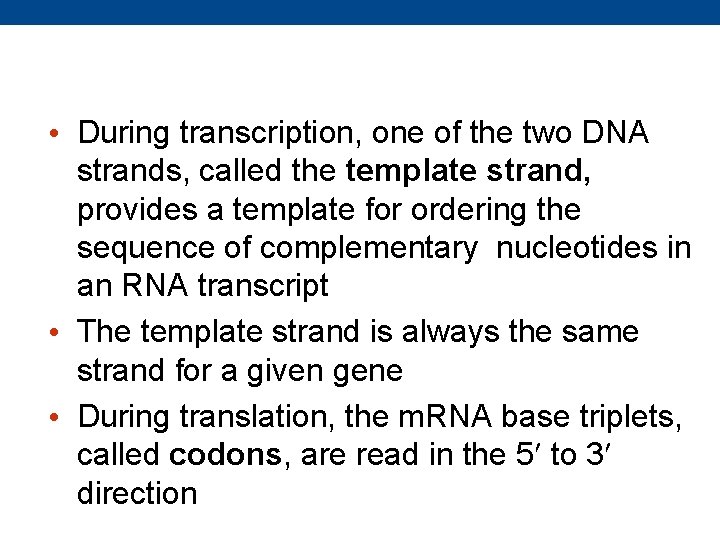
• During transcription, one of the two DNA strands, called the template strand, provides a template for ordering the sequence of complementary nucleotides in an RNA transcript • The template strand is always the same strand for a given gene • During translation, the m. RNA base triplets, called codons, are read in the 5 to 3 direction
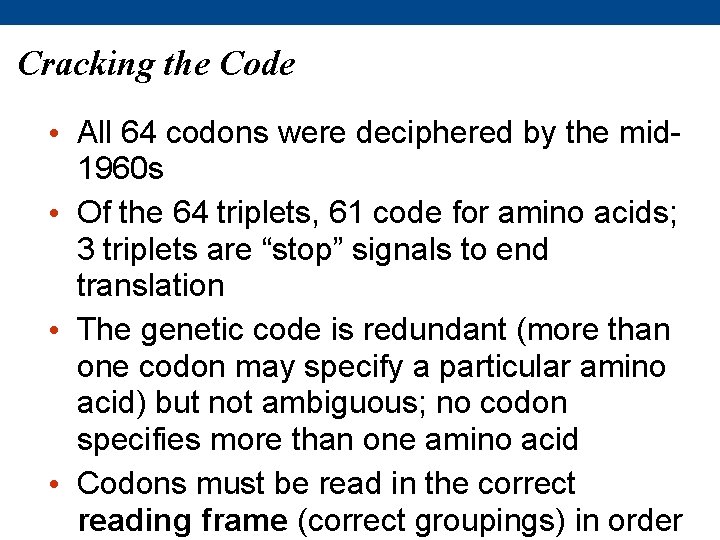
Cracking the Code • All 64 codons were deciphered by the mid 1960 s • Of the 64 triplets, 61 code for amino acids; 3 triplets are “stop” signals to end translation • The genetic code is redundant (more than one codon may specify a particular amino acid) but not ambiguous; no codon specifies more than one amino acid • Codons must be read in the correct reading frame (correct groupings) in order
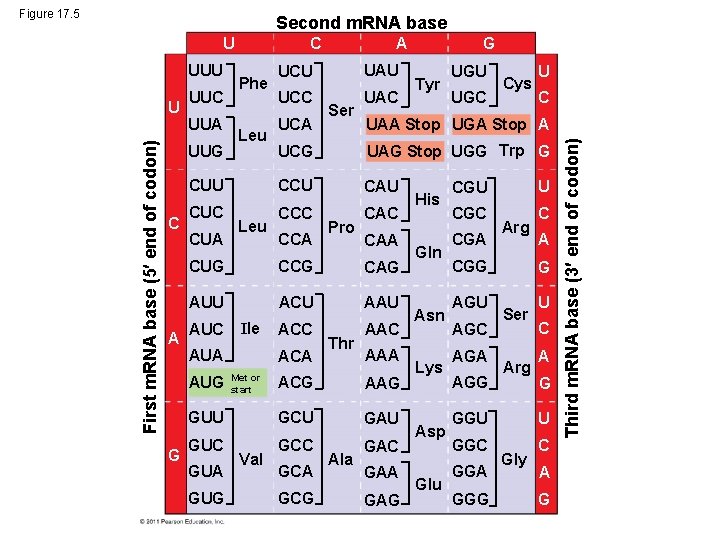
Figure 17. 5 Second m. RNA base UUU First m. RNA base (5 end of codon) U UUC UUA A Leu UCU UAU UCC UAC UCA Ser Tyr UGU UGC Cys U C UAA Stop UGA Stop A UAG Stop UGG Trp G CUU CCU CAU CUC CCC CAC CUA Leu CCA Pro CAA CUG CCG CAG AUU ACU AAU ACC AAC AUC Ile AUA AUG G Phe G UCG UUG C ACA Met or start Thr AAA ACG AAG GUU GCU GAU GUC GCC GAC GUA GUG Val GCA GCG Ala GAA GAG His Gln Asn Lys Asp Glu CGU U CGC C CGA Arg CGG AGU AGC AGA AGG A G Ser Arg U C A G GGU U GGC C GGA GGG Gly A G Third m. RNA base (3 end of codon) U
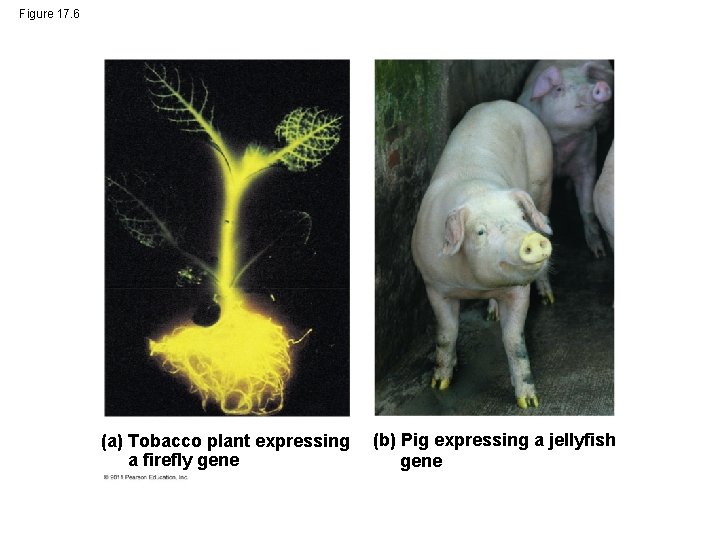
Figure 17. 6 (a) Tobacco plant expressing a firefly gene (b) Pig expressing a jellyfish gene
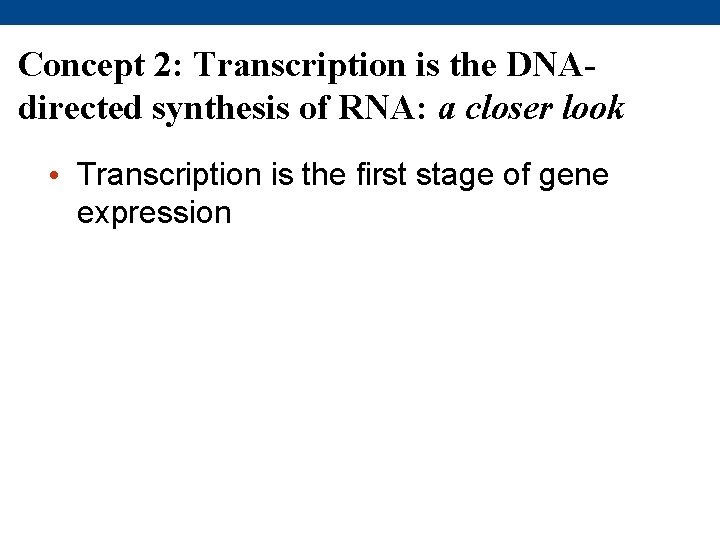
Concept 2: Transcription is the DNAdirected synthesis of RNA: a closer look • Transcription is the first stage of gene expression
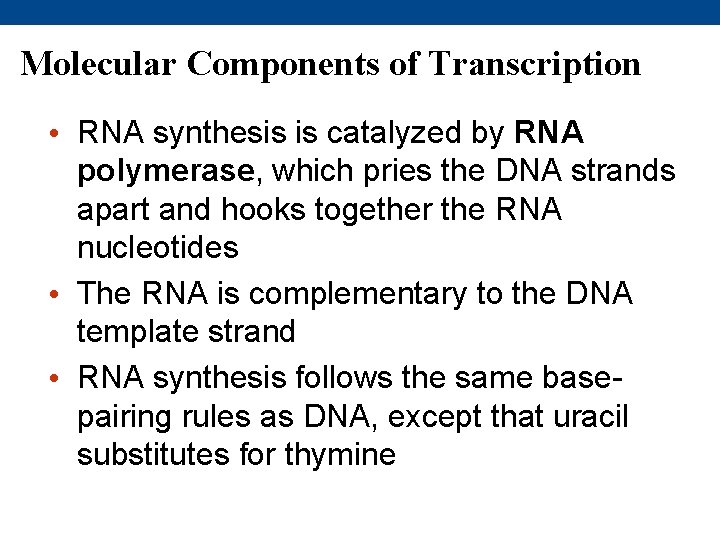
Molecular Components of Transcription • RNA synthesis is catalyzed by RNA polymerase, which pries the DNA strands apart and hooks together the RNA nucleotides • The RNA is complementary to the DNA template strand • RNA synthesis follows the same basepairing rules as DNA, except that uracil substitutes for thymine
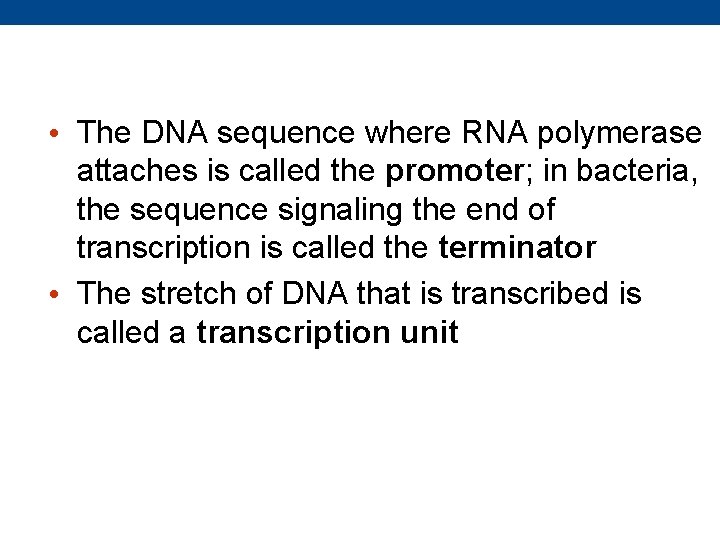
• The DNA sequence where RNA polymerase attaches is called the promoter; in bacteria, the sequence signaling the end of transcription is called the terminator • The stretch of DNA that is transcribed is called a transcription unit
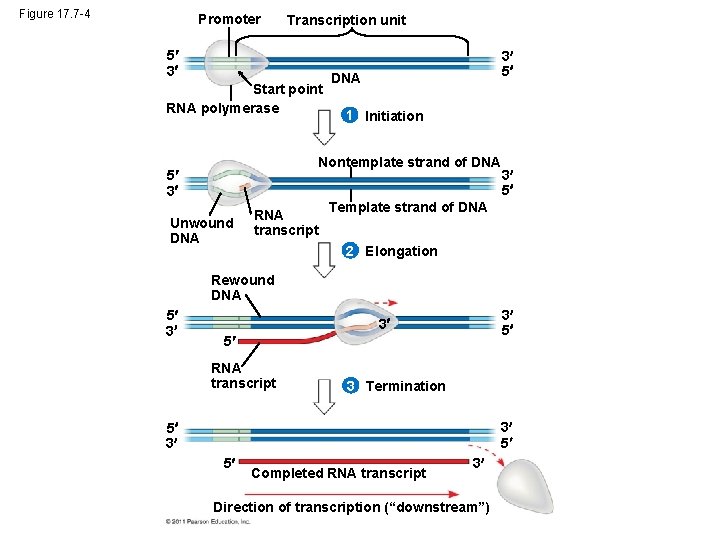
Figure 17. 7 -4 Promoter Transcription unit 5 3 Start point RNA polymerase 3 5 DNA 1 Initiation Nontemplate strand of DNA 5 3 Unwound DNA RNA transcript 3 5 Template strand of DNA 2 Elongation Rewound DNA 5 3 3 5 RNA transcript 3 Termination 3 5 5 3 5 Completed RNA transcript 3 Direction of transcription (“downstream”)
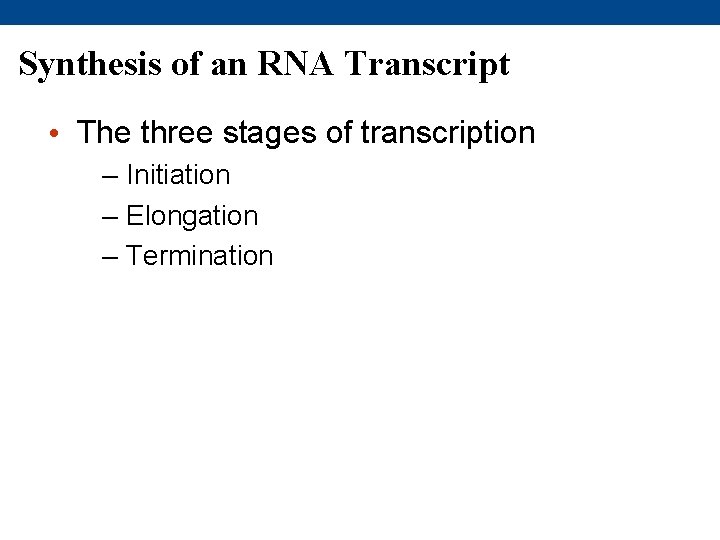
Synthesis of an RNA Transcript • The three stages of transcription – Initiation – Elongation – Termination
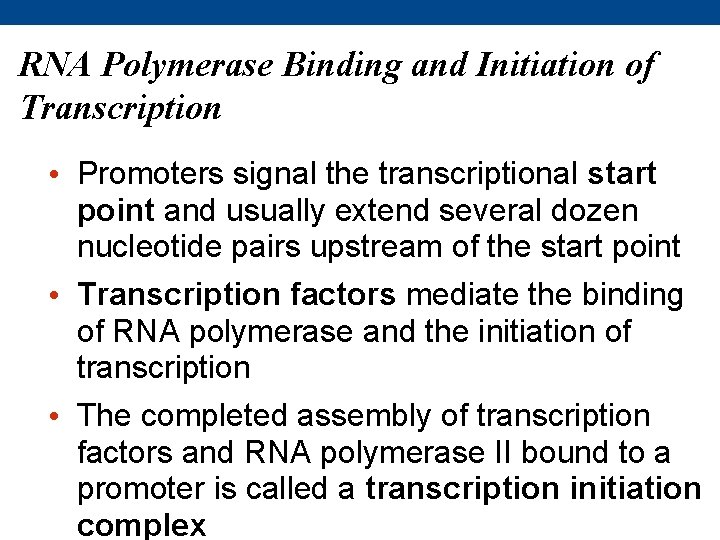
RNA Polymerase Binding and Initiation of Transcription • Promoters signal the transcriptional start point and usually extend several dozen nucleotide pairs upstream of the start point • Transcription factors mediate the binding of RNA polymerase and the initiation of transcription • The completed assembly of transcription factors and RNA polymerase II bound to a promoter is called a transcription initiation complex
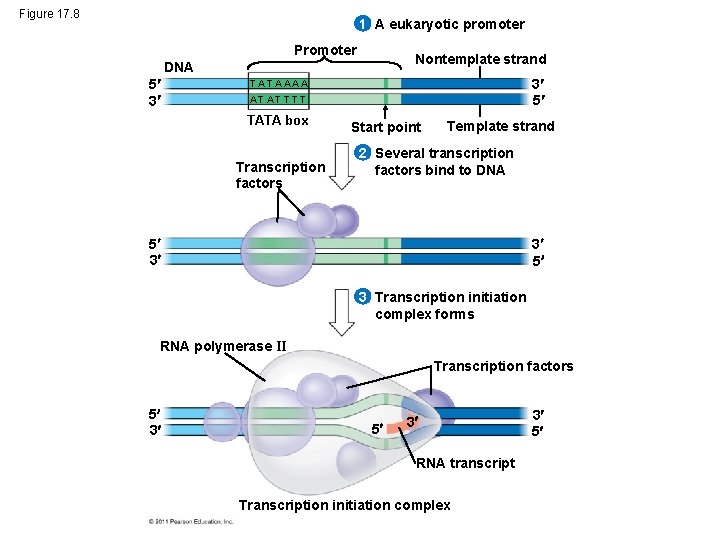
Figure 17. 8 1 A eukaryotic promoter Promoter Nontemplate strand DNA 5 3 3 5 T A A AA A T AT T TATA box Transcription factors Start point Template strand 2 Several transcription factors bind to DNA 5 3 3 5 3 Transcription initiation complex forms RNA polymerase II Transcription factors 5 3 5 3 RNA transcript Transcription initiation complex 3 5
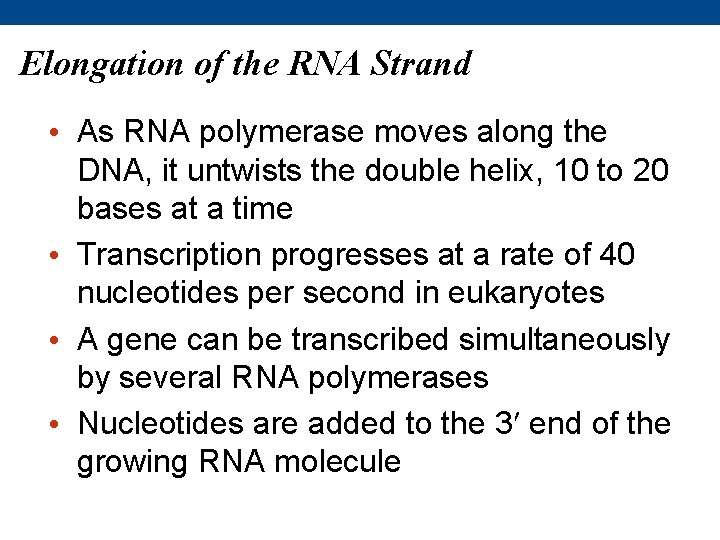
Elongation of the RNA Strand • As RNA polymerase moves along the DNA, it untwists the double helix, 10 to 20 bases at a time • Transcription progresses at a rate of 40 nucleotides per second in eukaryotes • A gene can be transcribed simultaneously by several RNA polymerases • Nucleotides are added to the 3 end of the growing RNA molecule
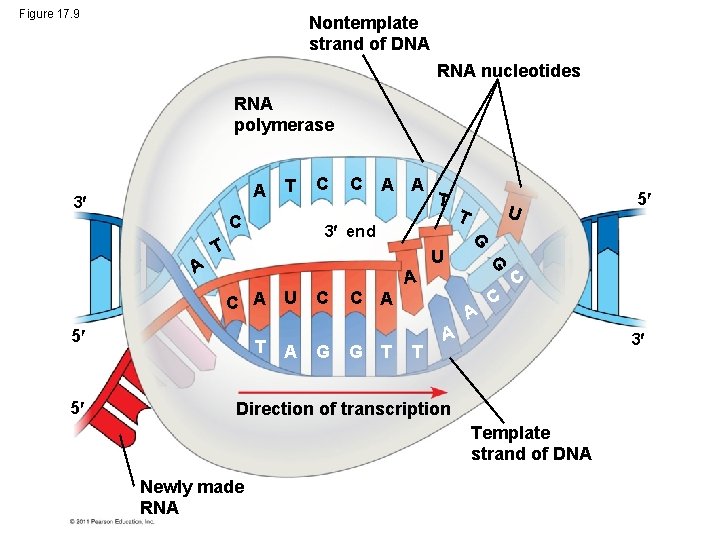
Figure 17. 9 Nontemplate strand of DNA RNA nucleotides RNA polymerase A 3 T C A A T 3 end T 5 T U A C G C A G T U T G U C A 5 C A A T C C A 3 Direction of transcription Template strand of DNA Newly made RNA 5 G A C
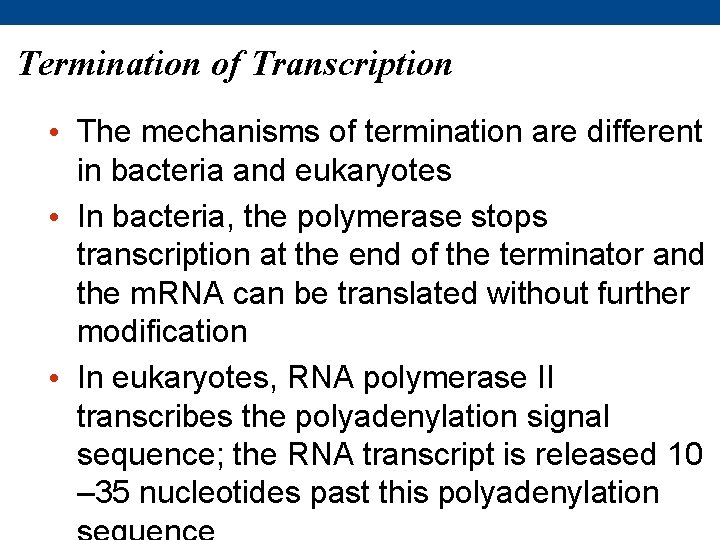
Termination of Transcription • The mechanisms of termination are different in bacteria and eukaryotes • In bacteria, the polymerase stops transcription at the end of the terminator and the m. RNA can be translated without further modification • In eukaryotes, RNA polymerase II transcribes the polyadenylation signal sequence; the RNA transcript is released 10 – 35 nucleotides past this polyadenylation
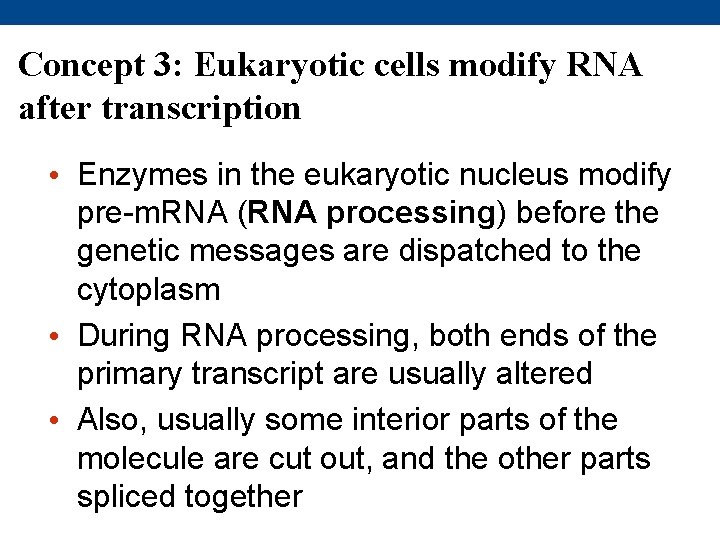
Concept 3: Eukaryotic cells modify RNA after transcription • Enzymes in the eukaryotic nucleus modify pre-m. RNA (RNA processing) before the genetic messages are dispatched to the cytoplasm • During RNA processing, both ends of the primary transcript are usually altered • Also, usually some interior parts of the molecule are cut out, and the other parts spliced together
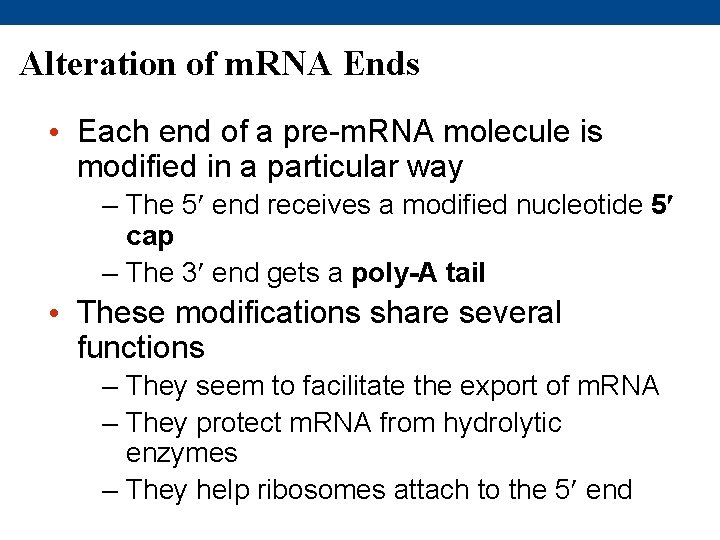
Alteration of m. RNA Ends • Each end of a pre-m. RNA molecule is modified in a particular way – The 5 end receives a modified nucleotide 5 cap – The 3 end gets a poly-A tail • These modifications share several functions – They seem to facilitate the export of m. RNA – They protect m. RNA from hydrolytic enzymes – They help ribosomes attach to the 5 end
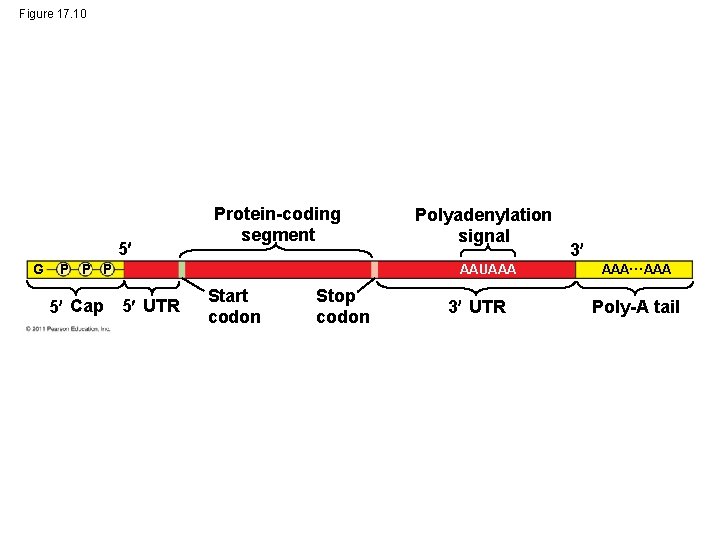
Figure 17. 10 5 G Protein-coding segment P P P 5 Cap 5 UTR Polyadenylation signal AAUAAA Start codon Stop codon 3 UTR 3 AAA… AAA Poly-A tail
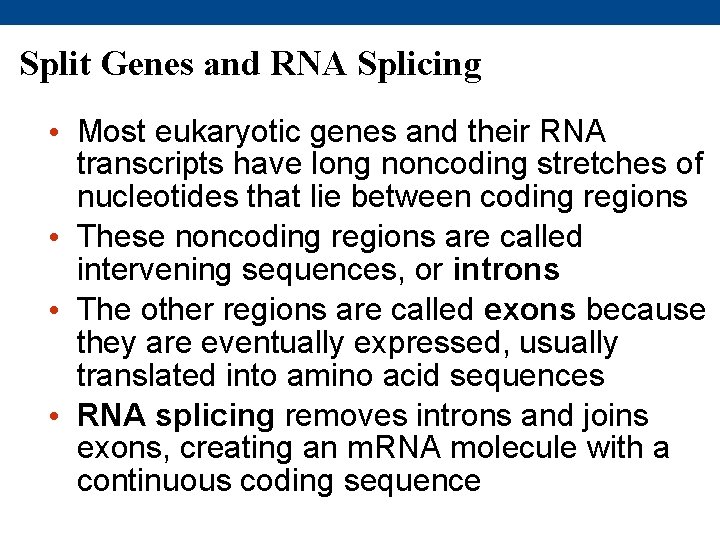
Split Genes and RNA Splicing • Most eukaryotic genes and their RNA transcripts have long noncoding stretches of nucleotides that lie between coding regions • These noncoding regions are called intervening sequences, or introns • The other regions are called exons because they are eventually expressed, usually translated into amino acid sequences • RNA splicing removes introns and joins exons, creating an m. RNA molecule with a continuous coding sequence
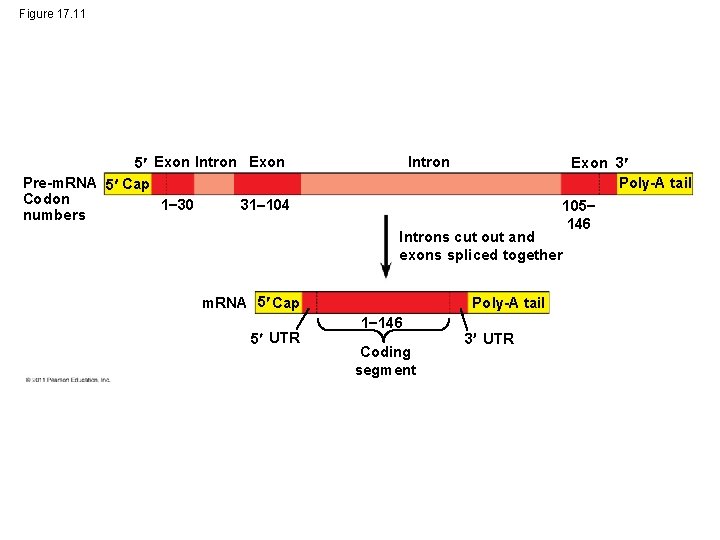
Figure 17. 11 5 Exon Intron Exon Pre-m. RNA 5 Cap Codon 1 30 31 104 numbers Intron Exon 3 Poly-A tail 105 146 Introns cut out and exons spliced together m. RNA 5 Cap 5 UTR Poly-A tail 1 146 Coding segment 3 UTR
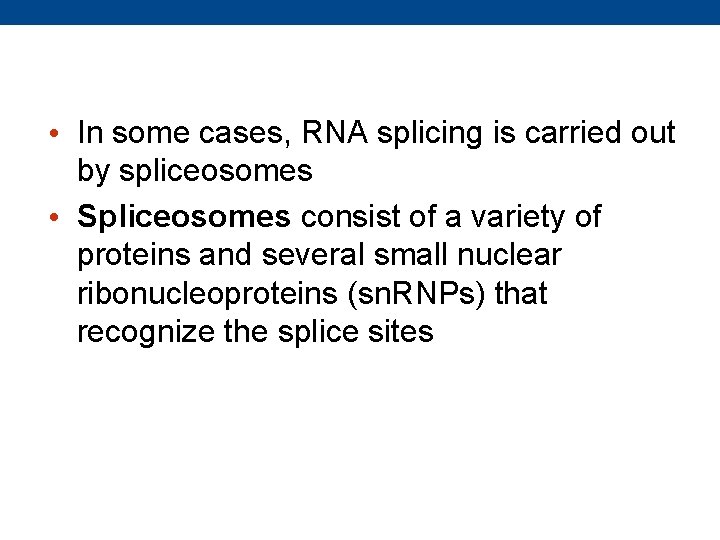
• In some cases, RNA splicing is carried out by spliceosomes • Spliceosomes consist of a variety of proteins and several small nuclear ribonucleoproteins (sn. RNPs) that recognize the splice sites
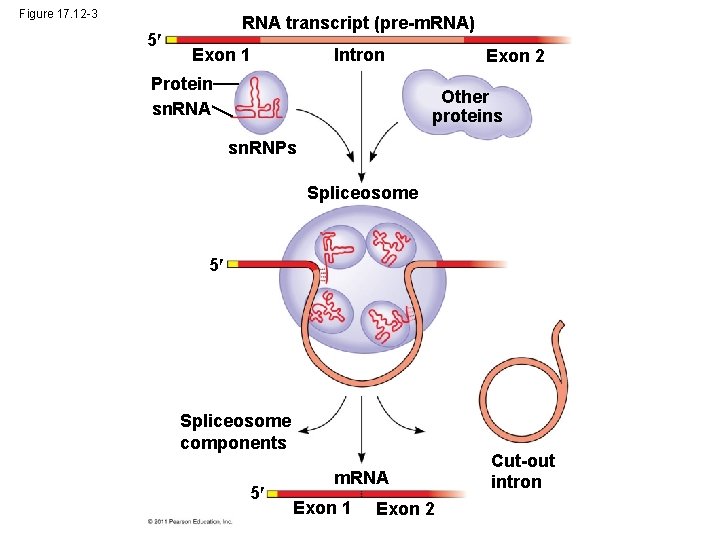
Figure 17. 12 -3 5 RNA transcript (pre-m. RNA) Exon 1 Intron Protein sn. RNA Exon 2 Other proteins sn. RNPs Spliceosome 5 Spliceosome components 5 m. RNA Exon 1 Exon 2 Cut-out intron
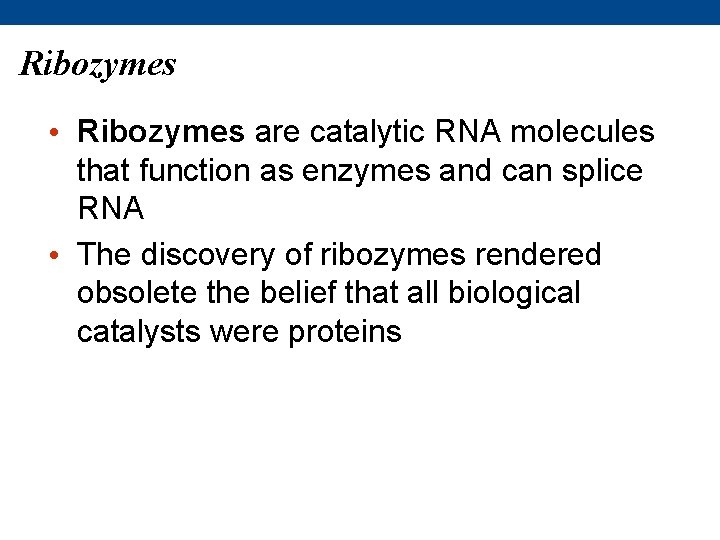
Ribozymes • Ribozymes are catalytic RNA molecules that function as enzymes and can splice RNA • The discovery of ribozymes rendered obsolete the belief that all biological catalysts were proteins
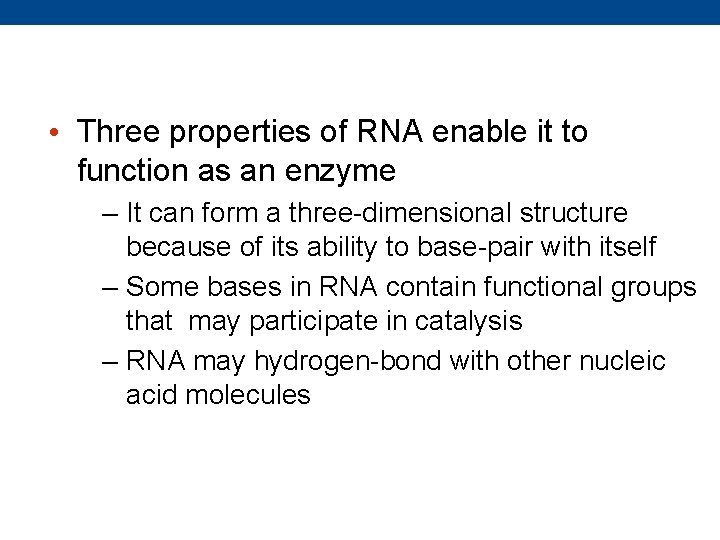
• Three properties of RNA enable it to function as an enzyme – It can form a three-dimensional structure because of its ability to base-pair with itself – Some bases in RNA contain functional groups that may participate in catalysis – RNA may hydrogen-bond with other nucleic acid molecules
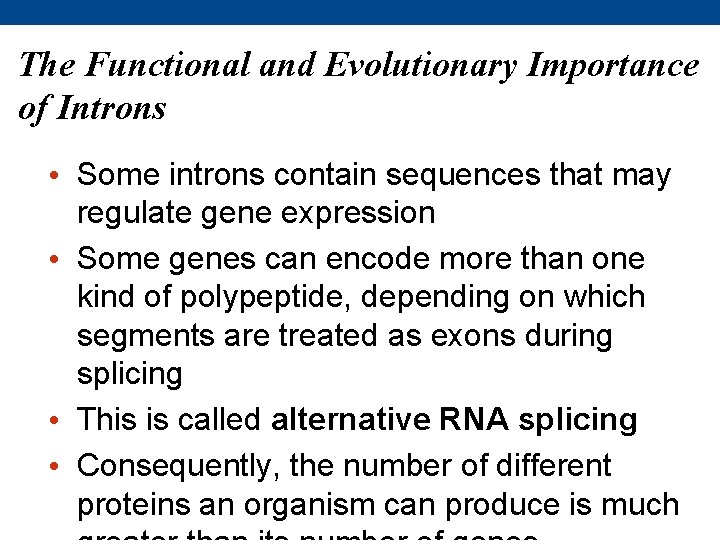
The Functional and Evolutionary Importance of Introns • Some introns contain sequences that may regulate gene expression • Some genes can encode more than one kind of polypeptide, depending on which segments are treated as exons during splicing • This is called alternative RNA splicing • Consequently, the number of different proteins an organism can produce is much
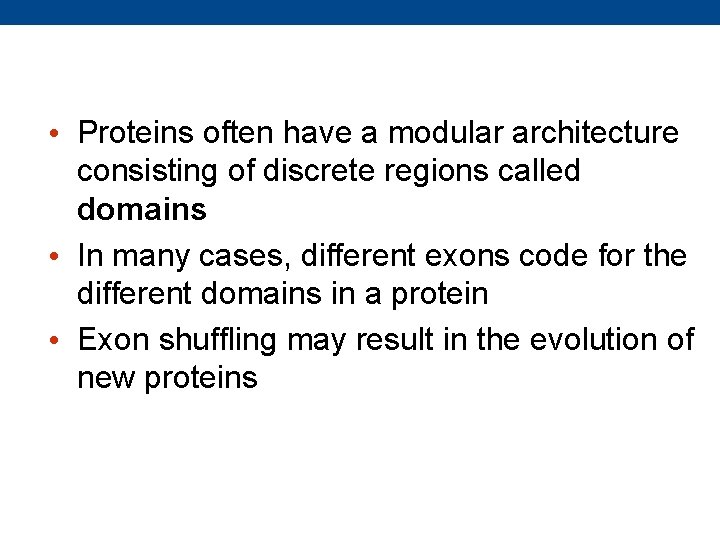
• Proteins often have a modular architecture consisting of discrete regions called domains • In many cases, different exons code for the different domains in a protein • Exon shuffling may result in the evolution of new proteins
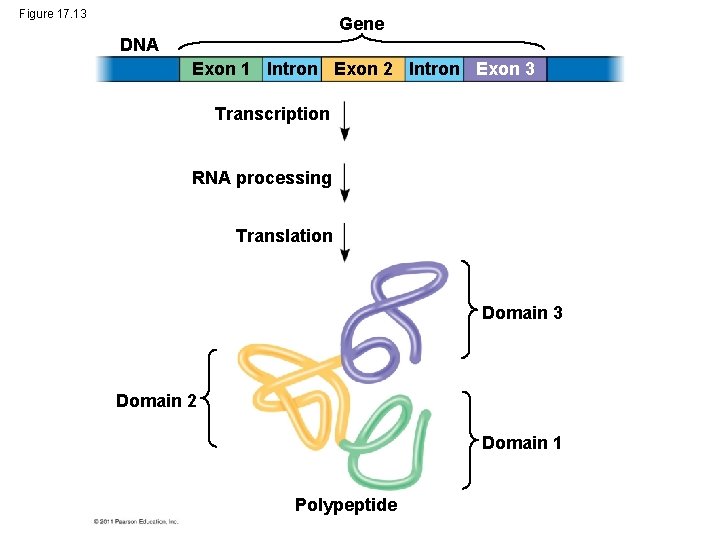
Figure 17. 13 Gene DNA Exon 1 Intron Exon 2 Intron Exon 3 Transcription RNA processing Translation Domain 3 Domain 2 Domain 1 Polypeptide
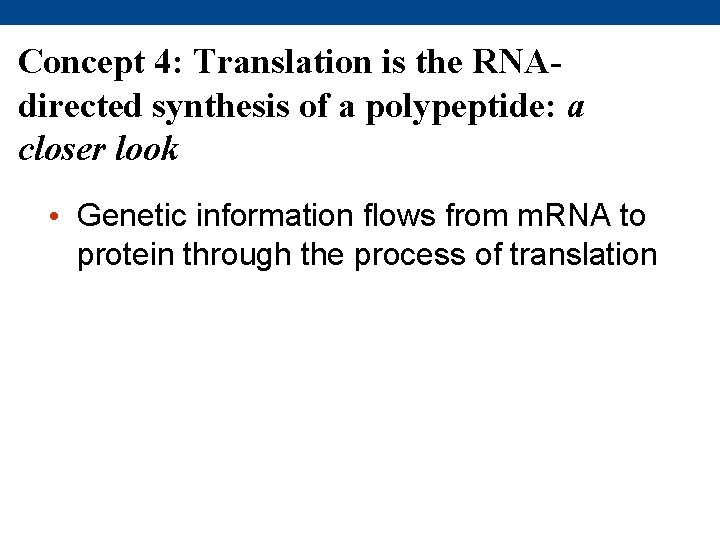
Concept 4: Translation is the RNAdirected synthesis of a polypeptide: a closer look • Genetic information flows from m. RNA to protein through the process of translation
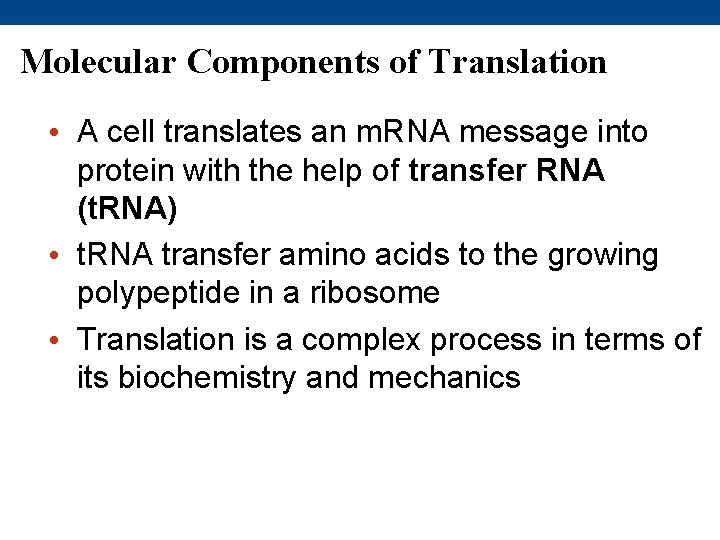
Molecular Components of Translation • A cell translates an m. RNA message into protein with the help of transfer RNA (t. RNA) • t. RNA transfer amino acids to the growing polypeptide in a ribosome • Translation is a complex process in terms of its biochemistry and mechanics
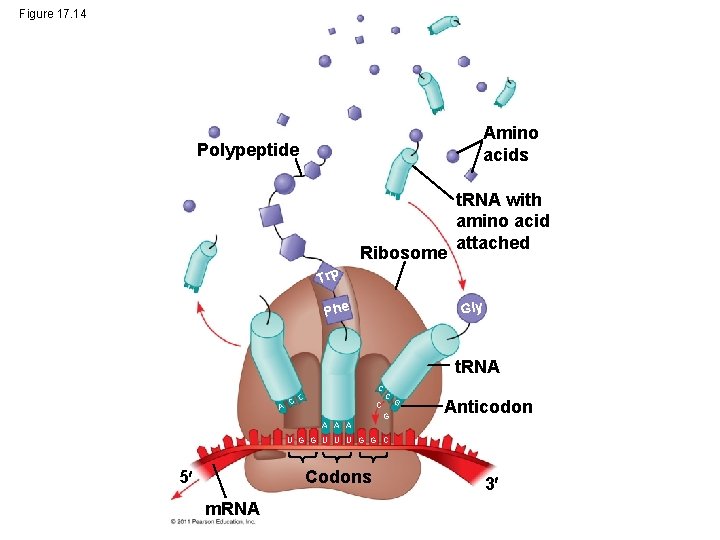
Figure 17. 14 Amino acids Polypeptide Ribosome t. RNA with amino acid attached Trp Gly Phe t. RNA C C C C G G Anticodon A A A U G G U U U G G C 5 Codons m. RNA 3
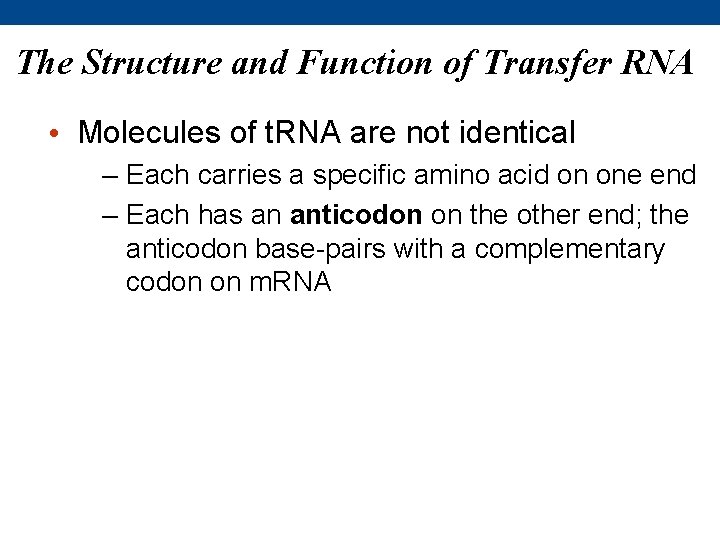
The Structure and Function of Transfer RNA • Molecules of t. RNA are not identical – Each carries a specific amino acid on one end – Each has an anticodon on the other end; the anticodon base-pairs with a complementary codon on m. RNA
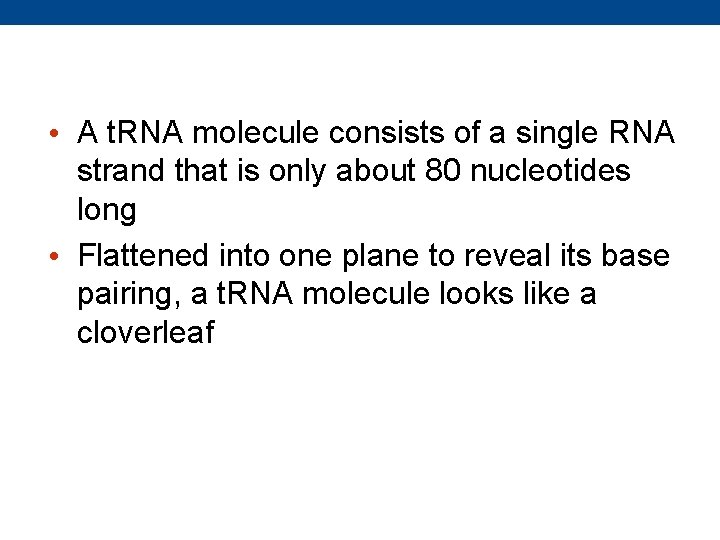
• A t. RNA molecule consists of a single RNA strand that is only about 80 nucleotides long • Flattened into one plane to reveal its base pairing, a t. RNA molecule looks like a cloverleaf
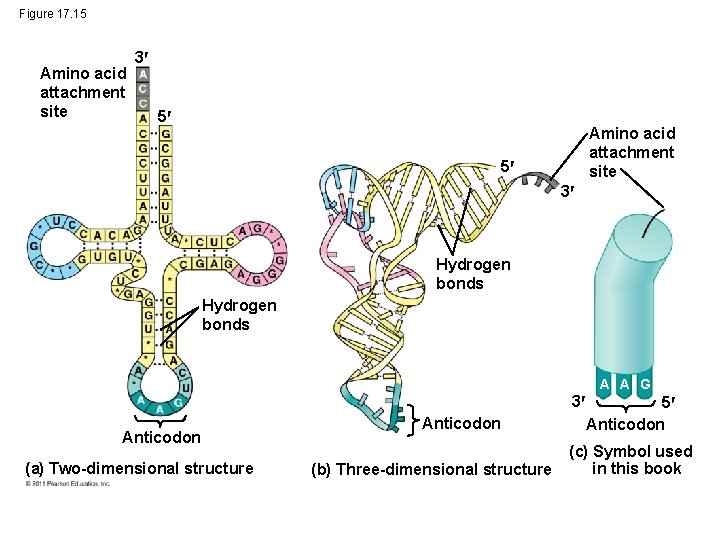
Figure 17. 15 Amino acid attachment site 3 5 Amino acid attachment site 5 3 Hydrogen bonds A A G 3 Anticodon (a) Two-dimensional structure Anticodon (b) Three-dimensional structure 5 Anticodon (c) Symbol used in this book
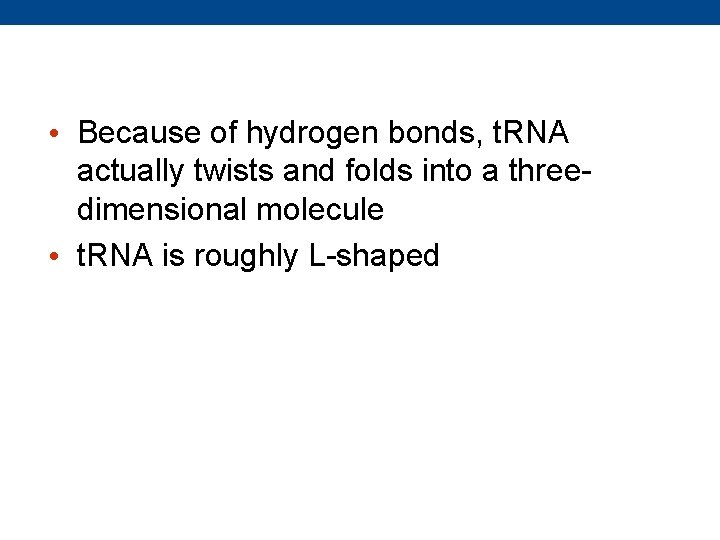
• Because of hydrogen bonds, t. RNA actually twists and folds into a threedimensional molecule • t. RNA is roughly L-shaped
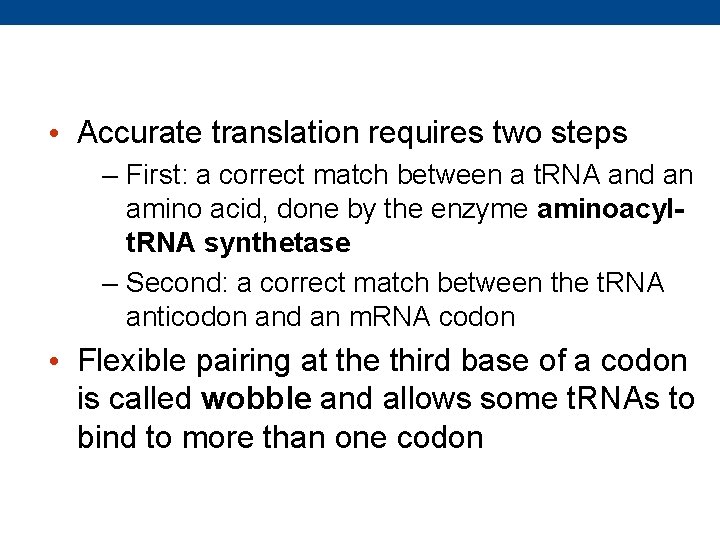
• Accurate translation requires two steps – First: a correct match between a t. RNA and an amino acid, done by the enzyme aminoacylt. RNA synthetase – Second: a correct match between the t. RNA anticodon and an m. RNA codon • Flexible pairing at the third base of a codon is called wobble and allows some t. RNAs to bind to more than one codon
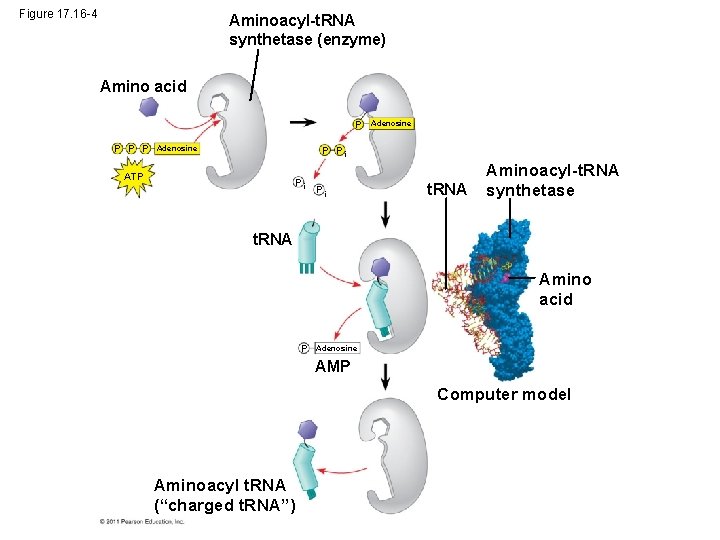
Figure 17. 16 -4 Aminoacyl-t. RNA synthetase (enzyme) Amino acid P P Adenosine P Pi ATP Pi Pi t. RNA Aminoacyl-t. RNA synthetase t. RNA Amino acid P Adenosine AMP Computer model Aminoacyl t. RNA (“charged t. RNA”)
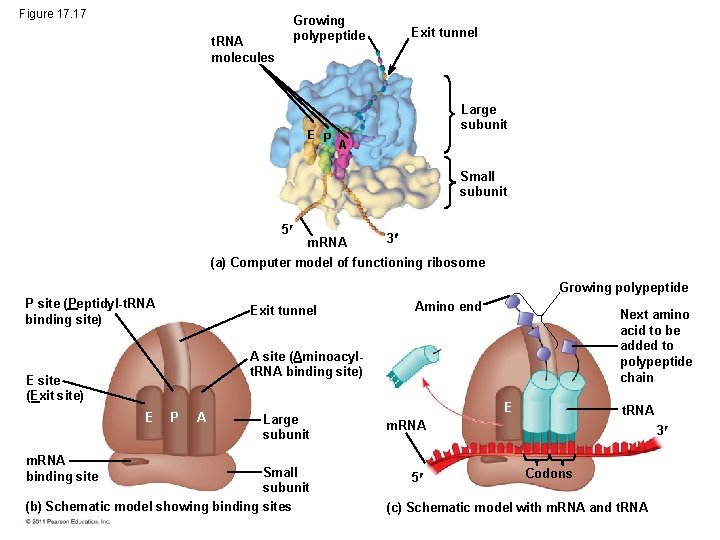
Figure 17. 17 Growing polypeptide t. RNA molecules E P Exit tunnel Large subunit A Small subunit 5 m. RNA 3 (a) Computer model of functioning ribosome Growing polypeptide P site (Peptidyl-t. RNA binding site) Exit tunnel Amino end A site (Aminoacylt. RNA binding site) E site (Exit site) E P A E Large subunit m. RNA Small subunit (b) Schematic model showing binding sites 5 m. RNA binding site Next amino acid to be added to polypeptide chain t. RNA 3 Codons (c) Schematic model with m. RNA and t. RNA
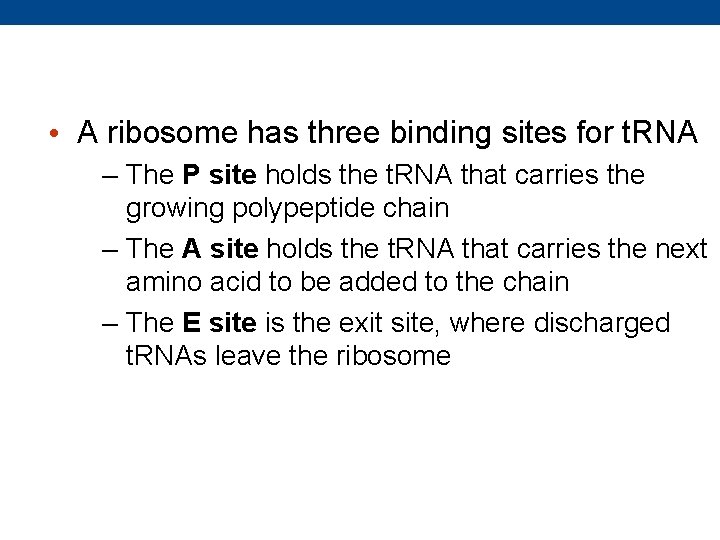
• A ribosome has three binding sites for t. RNA – The P site holds the t. RNA that carries the growing polypeptide chain – The A site holds the t. RNA that carries the next amino acid to be added to the chain – The E site is the exit site, where discharged t. RNAs leave the ribosome
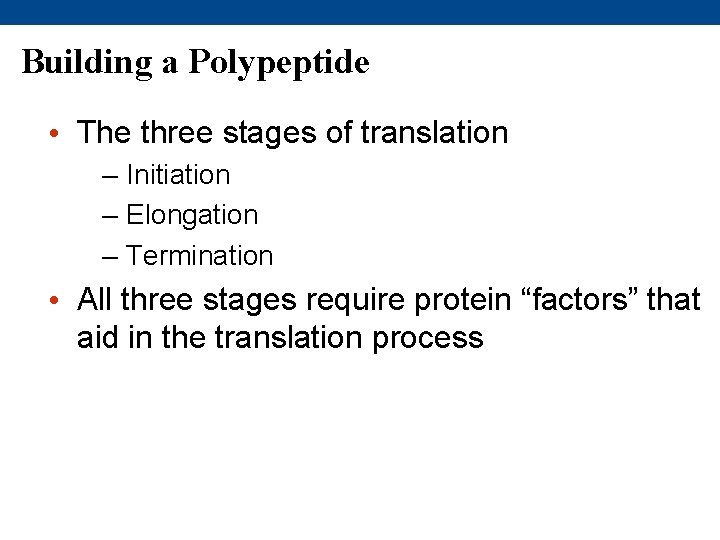
Building a Polypeptide • The three stages of translation – Initiation – Elongation – Termination • All three stages require protein “factors” that aid in the translation process
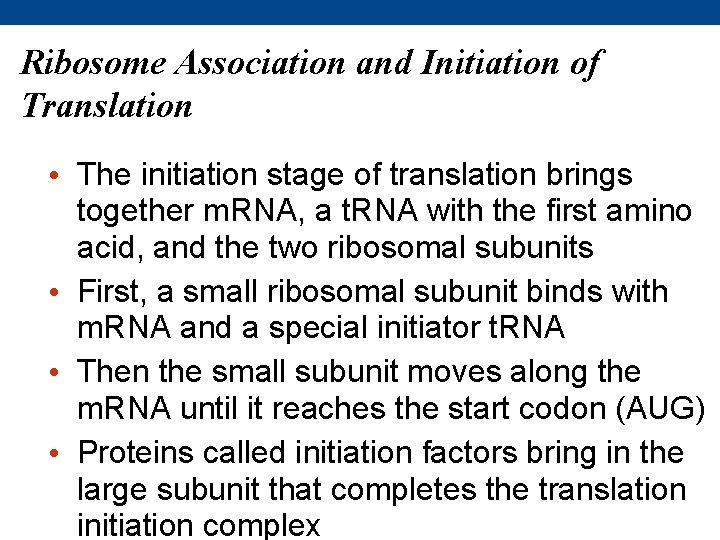
Ribosome Association and Initiation of Translation • The initiation stage of translation brings together m. RNA, a t. RNA with the first amino acid, and the two ribosomal subunits • First, a small ribosomal subunit binds with m. RNA and a special initiator t. RNA • Then the small subunit moves along the m. RNA until it reaches the start codon (AUG) • Proteins called initiation factors bring in the large subunit that completes the translation initiation complex
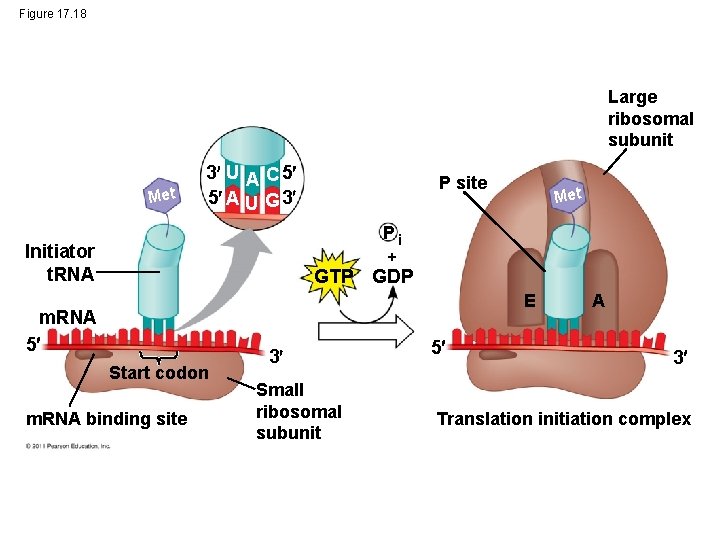
Figure 17. 18 Large ribosomal subunit Met 3 U A C 5 5 A U G 3 P site Met Pi Initiator t. RNA GTP GDP E m. RNA 5 Start codon m. RNA binding site 3 Small ribosomal subunit 5 A 3 Translation initiation complex
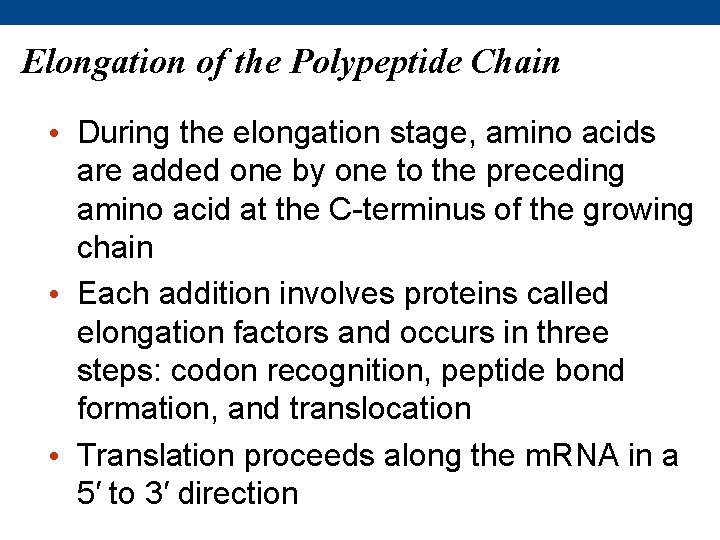
Elongation of the Polypeptide Chain • During the elongation stage, amino acids are added one by one to the preceding amino acid at the C-terminus of the growing chain • Each addition involves proteins called elongation factors and occurs in three steps: codon recognition, peptide bond formation, and translocation • Translation proceeds along the m. RNA in a 5′ to 3′ direction
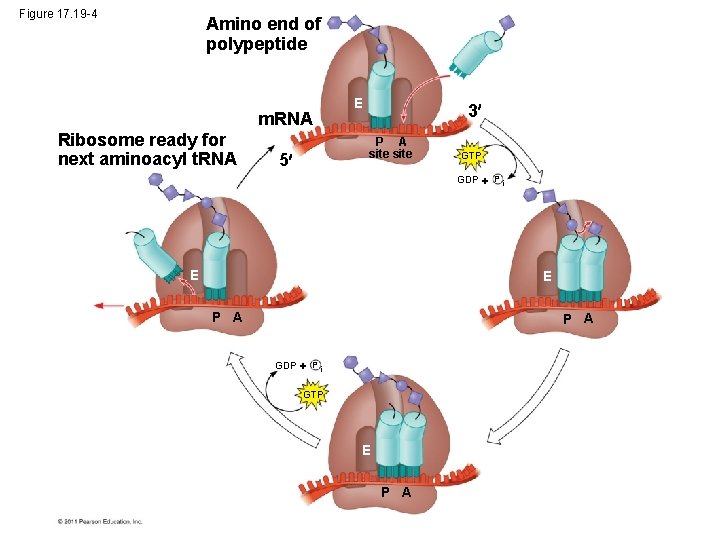
Figure 17. 19 -4 Amino end of polypeptide m. RNA Ribosome ready for next aminoacyl t. RNA E 3 P A site 5 GTP GDP P i E E P A GDP P i GTP E P A
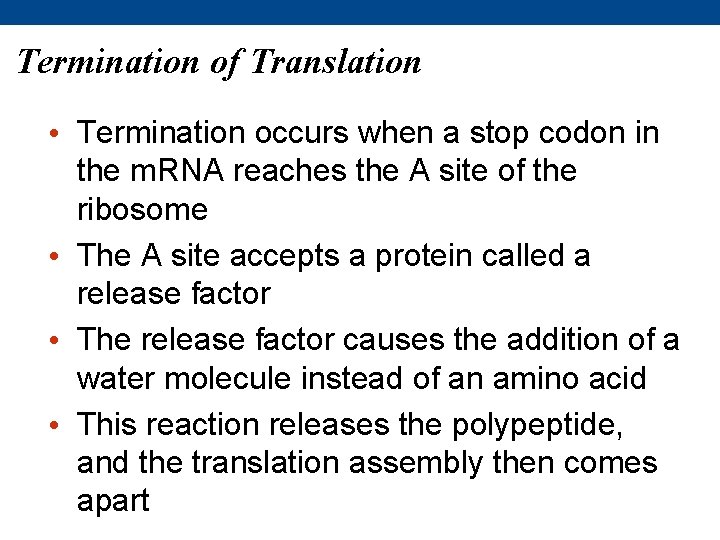
Termination of Translation • Termination occurs when a stop codon in the m. RNA reaches the A site of the ribosome • The A site accepts a protein called a release factor • The release factor causes the addition of a water molecule instead of an amino acid • This reaction releases the polypeptide, and the translation assembly then comes apart
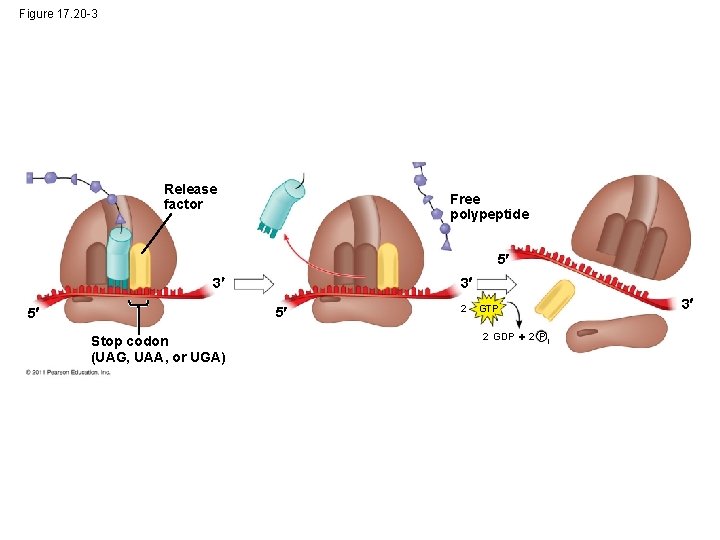
Figure 17. 20 -3 Release factor Free polypeptide 5 3 3 5 5 Stop codon (UAG, UAA, or UGA) 2 GTP 2 GDP 2 P i 3
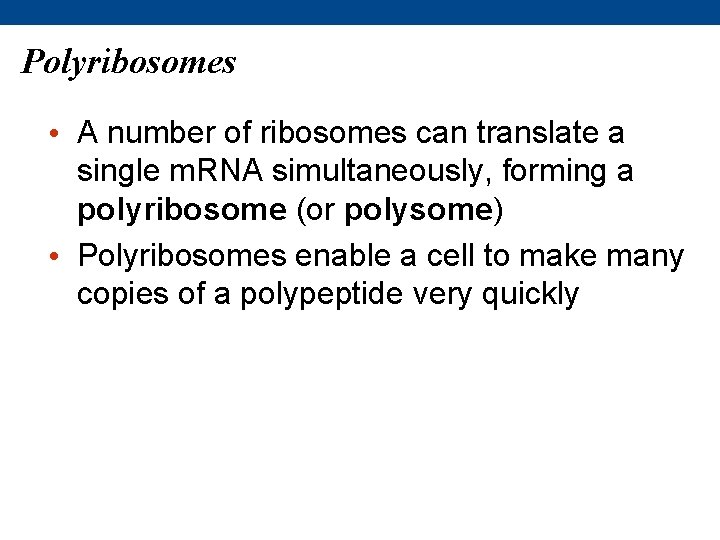
Polyribosomes • A number of ribosomes can translate a single m. RNA simultaneously, forming a polyribosome (or polysome) • Polyribosomes enable a cell to make many copies of a polypeptide very quickly
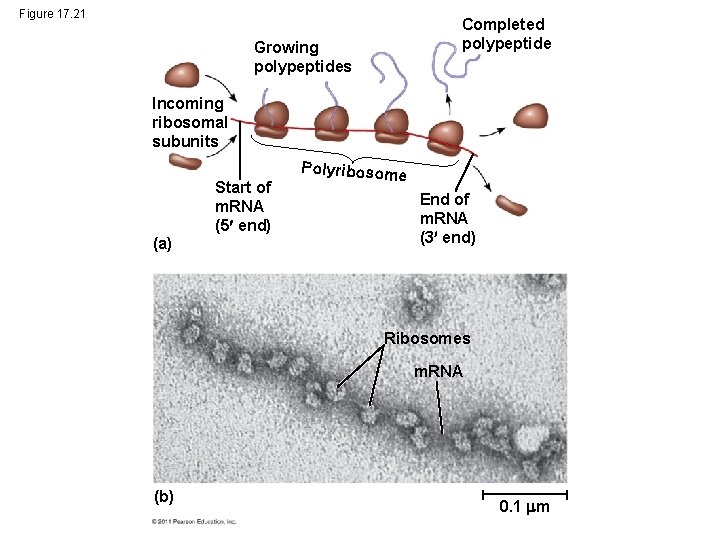
Figure 17. 21 Completed polypeptide Growing polypeptides Incoming ribosomal subunits (a) Start of m. RNA (5 end) Polyribosome End of m. RNA (3 end) Ribosomes m. RNA (b) 0. 1 m
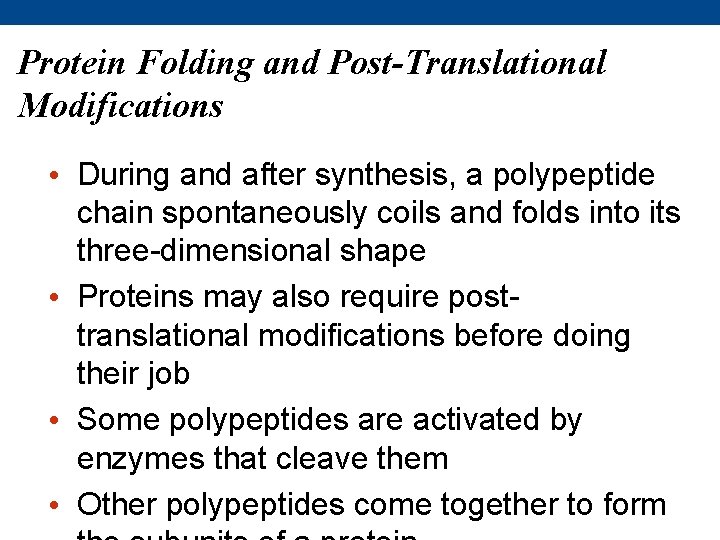
Protein Folding and Post-Translational Modifications • During and after synthesis, a polypeptide chain spontaneously coils and folds into its three-dimensional shape • Proteins may also require posttranslational modifications before doing their job • Some polypeptides are activated by enzymes that cleave them • Other polypeptides come together to form
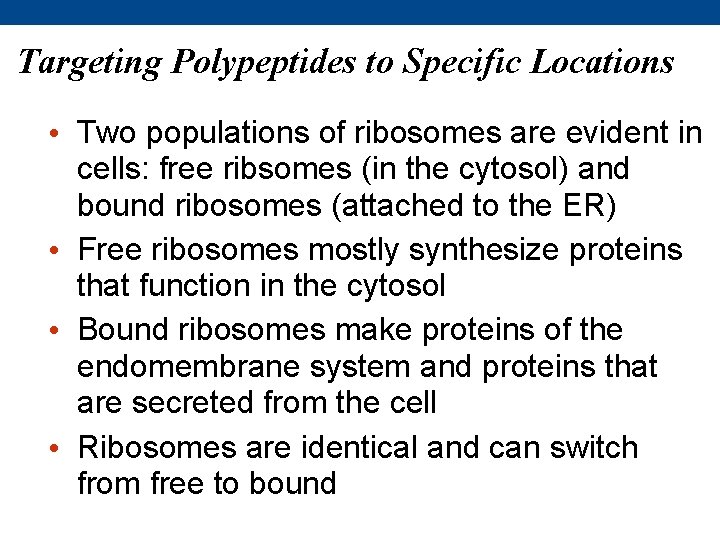
Targeting Polypeptides to Specific Locations • Two populations of ribosomes are evident in cells: free ribsomes (in the cytosol) and bound ribosomes (attached to the ER) • Free ribosomes mostly synthesize proteins that function in the cytosol • Bound ribosomes make proteins of the endomembrane system and proteins that are secreted from the cell • Ribosomes are identical and can switch from free to bound
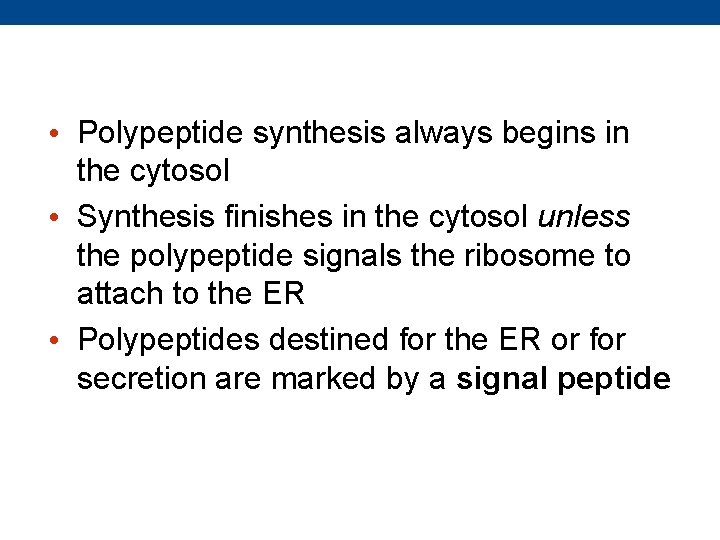
• Polypeptide synthesis always begins in the cytosol • Synthesis finishes in the cytosol unless the polypeptide signals the ribosome to attach to the ER • Polypeptides destined for the ER or for secretion are marked by a signal peptide
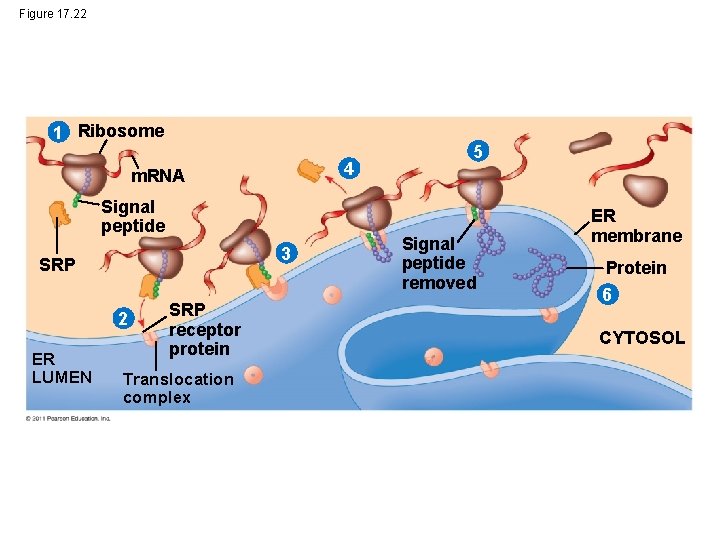
Figure 17. 22 1 Ribosome 4 m. RNA Signal peptide 3 SRP 2 ER LUMEN SRP receptor protein Translocation complex 5 Signal peptide removed ER membrane Protein 6 CYTOSOL
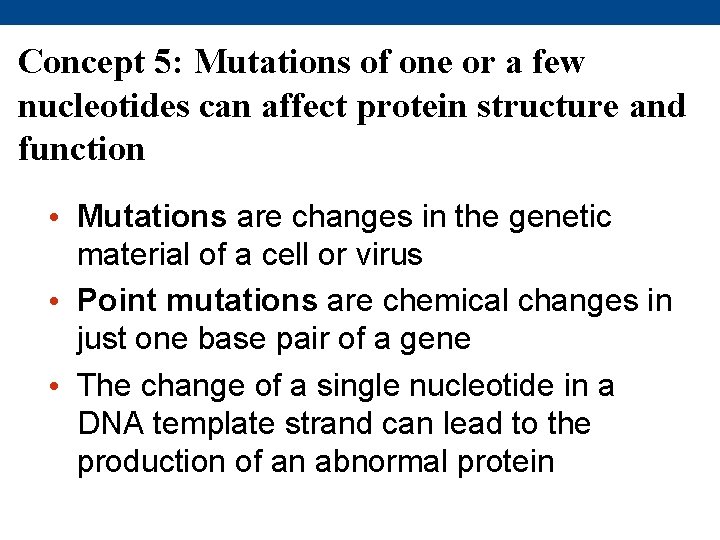
Concept 5: Mutations of one or a few nucleotides can affect protein structure and function • Mutations are changes in the genetic material of a cell or virus • Point mutations are chemical changes in just one base pair of a gene • The change of a single nucleotide in a DNA template strand can lead to the production of an abnormal protein
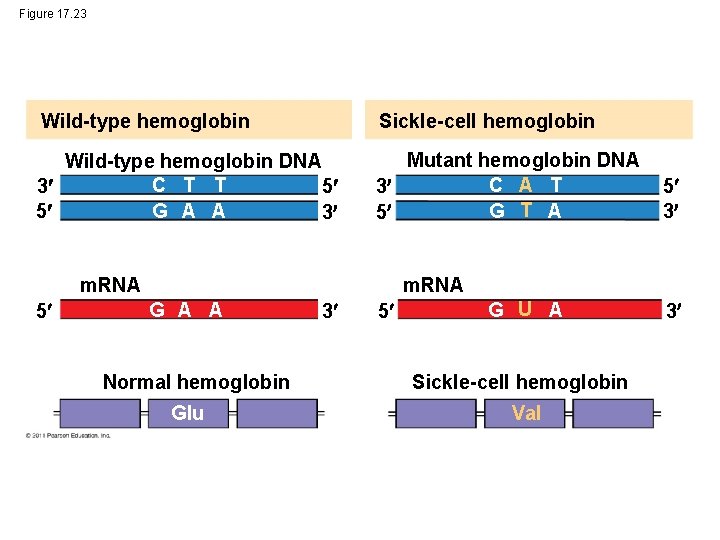
Figure 17. 23 Wild-type hemoglobin Sickle-cell hemoglobin Wild-type hemoglobin DNA C T T 3 5 G A A 5 3 Mutant hemoglobin DNA C A T 3 G T A 5 m. RNA 5 5 3 m. RNA G A A Normal hemoglobin Glu 3 5 G U A Sickle-cell hemoglobin Val 3
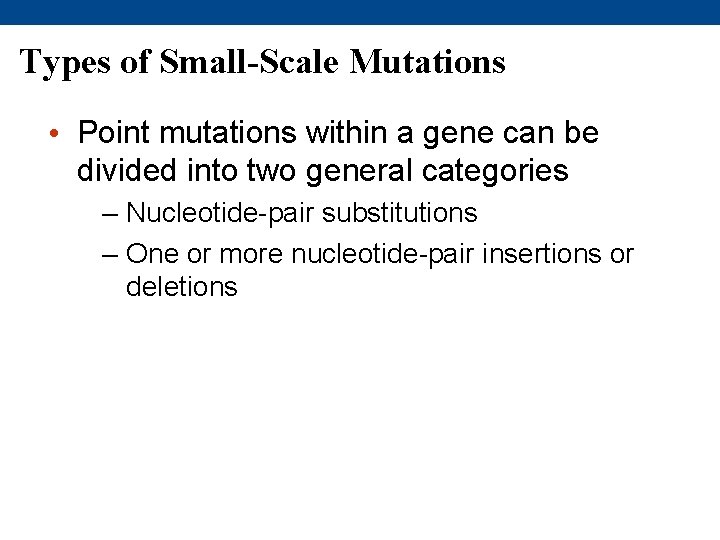
Types of Small-Scale Mutations • Point mutations within a gene can be divided into two general categories – Nucleotide-pair substitutions – One or more nucleotide-pair insertions or deletions
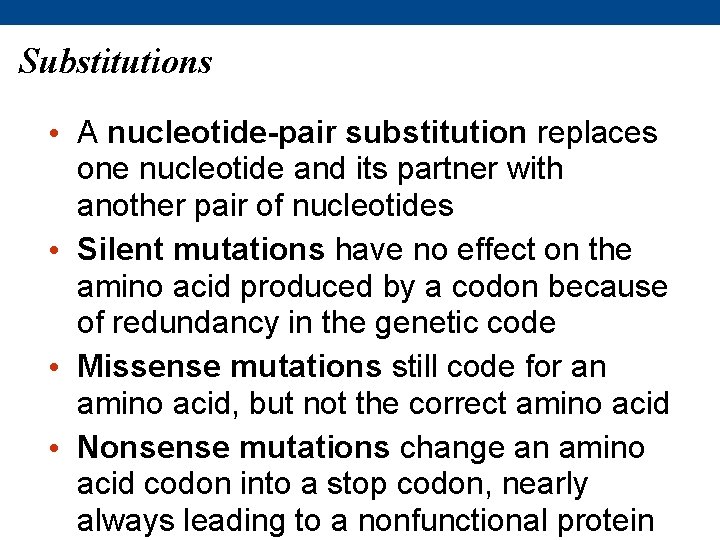
Substitutions • A nucleotide-pair substitution replaces one nucleotide and its partner with another pair of nucleotides • Silent mutations have no effect on the amino acid produced by a codon because of redundancy in the genetic code • Missense mutations still code for an amino acid, but not the correct amino acid • Nonsense mutations change an amino acid codon into a stop codon, nearly always leading to a nonfunctional protein
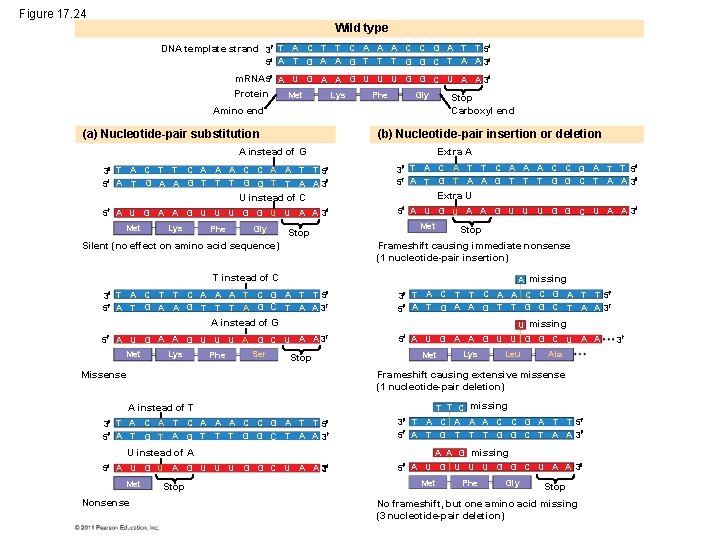
Figure 17. 24 Wild type DNA template strand 3 T A C T T C A A A C C G A T T 5 5 A T G A A G T T T G G C T A A 3 m. RNA 5 A U G A A G U U U G G C U A A 3 Protein Met Lys Phe Gly Stop Carboxyl end Amino end (b) Nucleotide-pair insertion or deletion (a) Nucleotide-pair substitution Extra A A instead of G 3 T A C T T C A A A C C A A T T 5 5 A T G A A G T T T G G T T A A 3 3 T A C A T T C A A A C C G A T T 5 5 A T G T A A G T T T G G C T A A 3 Extra U U instead of C 5 A U G A A G U U U G G U U A A 3 Met Lys Phe Gly Stop Silent (no effect on amino acid sequence) 5 A U G U A A G U U U G G C U A A 3 Met Stop Frameshift causing immediate nonsense (1 nucleotide-pair insertion) T instead of C A missing 3 T A C T T C A A A T C G A T T 5 5 A T G A A G T T T A G C T A A 3 3 T A C T T C A A C C G A T T 5 T 5 A T G A A G T T G G C T A A 3 A A instead of G U missing 5 A U G A A G U U U A G C U A A 3 Met Lys Phe Ser Stop Missense 5 A U G A A G U U G G C U A A Met Lys Leu Ala Frameshift causing extensive missense (1 nucleotide-pair deletion) A instead of T 3 T A C A T C A A A C C G A T T 5 5 A T G T A G T T T G G C T A A 3 U instead of A 5 A U G U A G U U U G G C U A A 3 Met Nonsense Stop T T C missing 3 T A C A A A C C G A T T 5 5 A T G T T T G G C T A A 3 A A G missing A A 5 A U G U U U G G C U A A 3 U Met Phe Gly Stop No frameshift, but one amino acid missing (3 nucleotide-pair deletion) 3
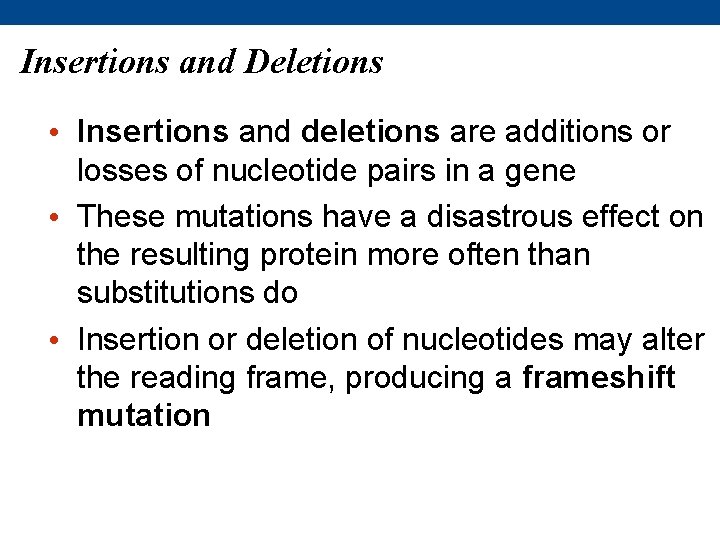
Insertions and Deletions • Insertions and deletions are additions or losses of nucleotide pairs in a gene • These mutations have a disastrous effect on the resulting protein more often than substitutions do • Insertion or deletion of nucleotides may alter the reading frame, producing a frameshift mutation
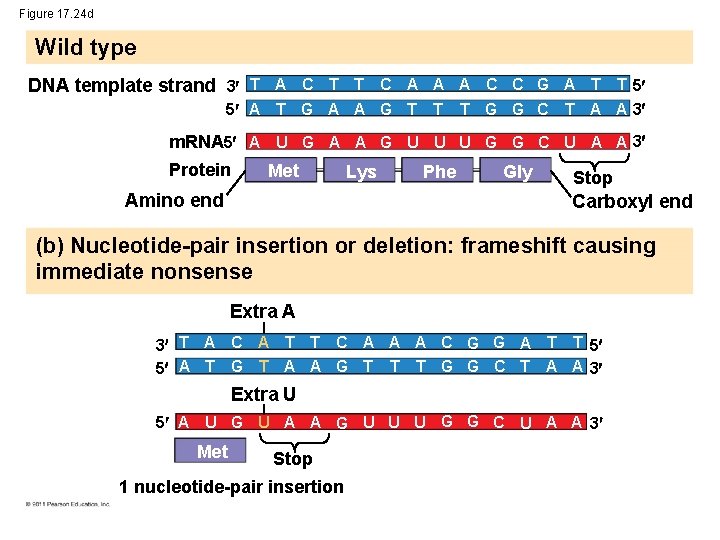
Figure 17. 24 d Wild type DNA template strand 3 T A C T T C A A A C C G A T T 5 5 A T G A A G T T T G G C T A A 3 m. RNA 5 A U G A A G U U U G G C U A A 3 Protein Met Amino end Lys Phe Gly Stop Carboxyl end (b) Nucleotide-pair insertion or deletion: frameshift causing immediate nonsense Extra A 3 T A C A T T C A A A C G G A T T 5 5 A T G T A A G T T T G G C T A A 3 Extra U 5 A U G U A A G U U U G G C U A A 3 Met Stop 1 nucleotide-pair insertion
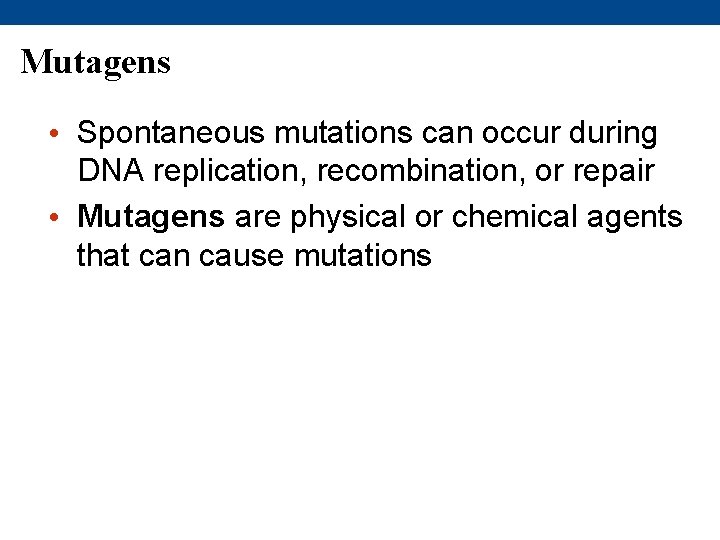
Mutagens • Spontaneous mutations can occur during DNA replication, recombination, or repair • Mutagens are physical or chemical agents that can cause mutations
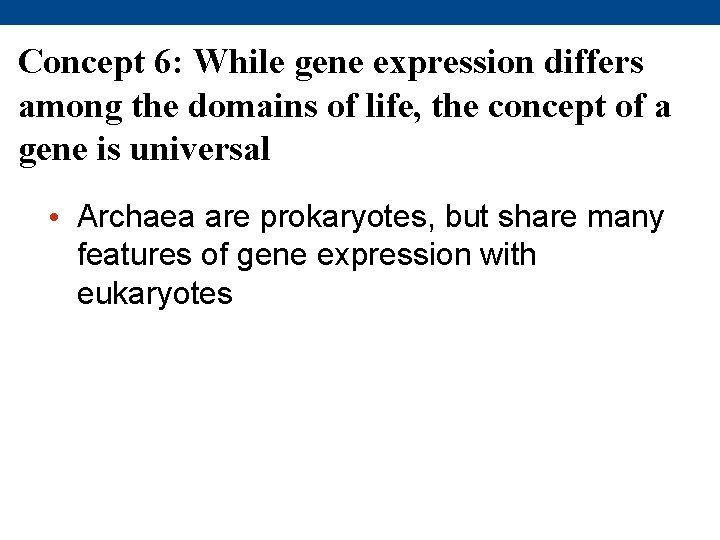
Concept 6: While gene expression differs among the domains of life, the concept of a gene is universal • Archaea are prokaryotes, but share many features of gene expression with eukaryotes
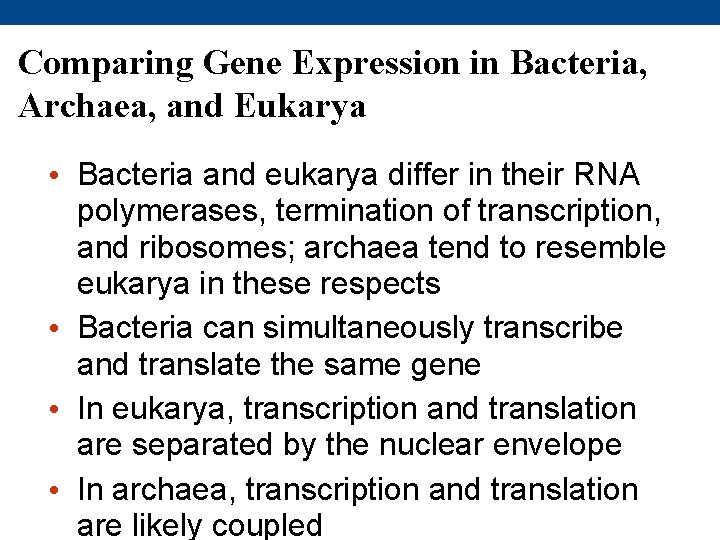
Comparing Gene Expression in Bacteria, Archaea, and Eukarya • Bacteria and eukarya differ in their RNA polymerases, termination of transcription, and ribosomes; archaea tend to resemble eukarya in these respects • Bacteria can simultaneously transcribe and translate the same gene • In eukarya, transcription and translation are separated by the nuclear envelope • In archaea, transcription and translation are likely coupled
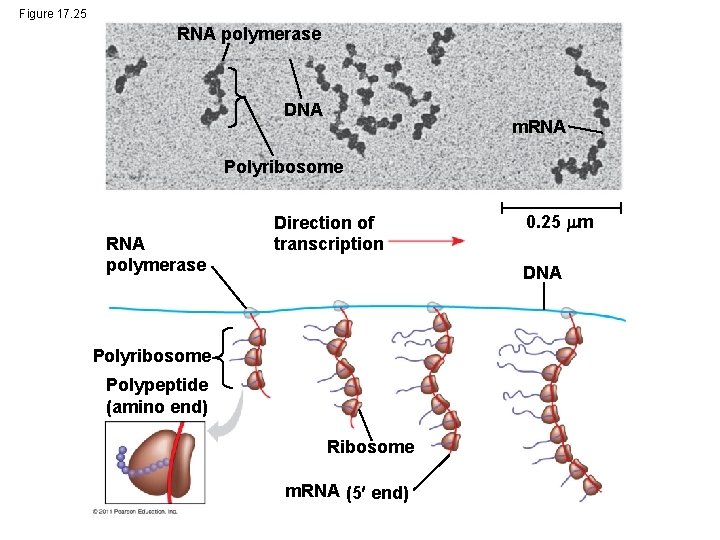
Figure 17. 25 RNA polymerase DNA m. RNA Polyribosome RNA polymerase Direction of transcription 0. 25 m DNA Polyribosome Polypeptide (amino end) Ribosome m. RNA (5 end)
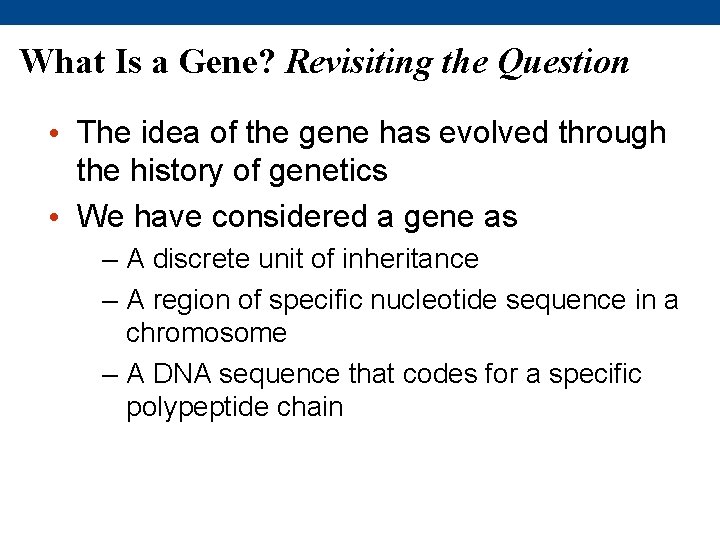
What Is a Gene? Revisiting the Question • The idea of the gene has evolved through the history of genetics • We have considered a gene as – A discrete unit of inheritance – A region of specific nucleotide sequence in a chromosome – A DNA sequence that codes for a specific polypeptide chain
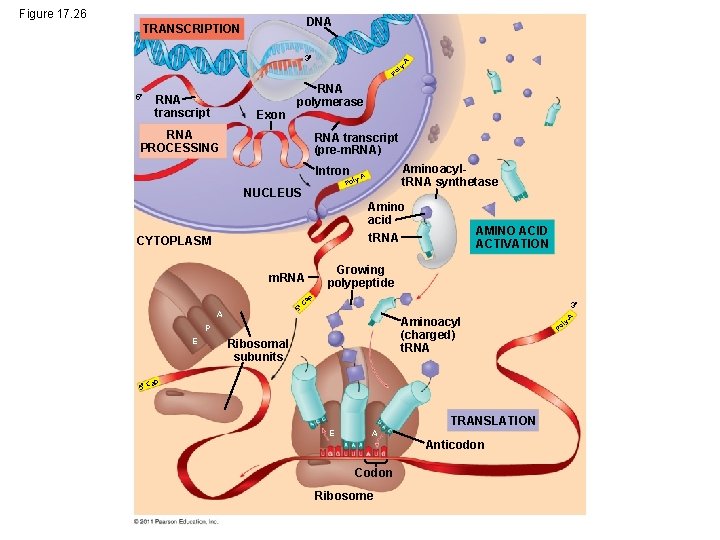
Figure 17. 26 DNA TRANSCRIPTION Po ly - A 3 5 RNA transcript Exon RNA polymerase RNA PROCESSING RNA transcript (pre-m. RNA) Intron Aminoacylt. RNA synthetase y-A Pol NUCLEUS Amino acid AMINO ACID ACTIVATION t. RNA CYTOPLASM Growing polypeptide ap m. RNA 3 5 C A Aminoacyl (charged) t. RNA P E Ribosomal subunits p a 5 C TRANSLATION E A Anticodon Codon Ribosome Po l A y-